Introduction
Every year, a huge number of teeth are lost due to dental caries, trauma or periodontal diseases. Regenerating lost teeth or their components is a dream for every dental professional. Tissue engineering is an emerging field of medicine which is essentially based on combining stem cells, growth factors and scaffolds for regenerating diseased or lost tissues. The contribution of scaffolds in tissue regeneration is indispensable as they serve as carriers to facilitate delivery of stem cells and/or growth factors at a local receptor site.
Scaffold biomaterials can be classified as natural or synthetic, rigid or non rigid and degradable or non degradable. Polymers are the most extensively used biomaterials for constructing scaffolds and their differential characteristics are attributed to the differences in their composition, structure and arrangement of constituent macromolecules. Researches currently are primarily directed towards tailoring the design characteristics of biomaterials such that precisely guided, coordinated, well timed and spatial interactions between the epithelial mesenchymal stem cells and scaffold matrix are encouraged. An overview of different types of biomaterials used for scaffold based tooth regeneration and application of nanotechnology in designing bioactive scaffolds shall be discussed in this article.
Strategies for Tooth Regeneration
Dental tissue regeneration aims at (a) regenerating an entire tooth which is structurally and functionally sound, (b) regenerating individual components of a tooth like enamel, dentin, pulp, cementum, periodontal ligament and alveolar bone. Bio engineered teeth, similar to natural teeth, are expected to occlude precisely in the dentition, establish proper contacts with adjacent teeth, provide proprioception, transmit masticatory loads and restore esthetics. To generate such teeth with predetermined morphology, it is highly important to precisely and orderly orient epithelial mesenchymal cell layers onto the scaffold as well as guide their interaction with the extracellular matrix. This differential placement of cells and their interaction with the matrix can possibly be achieved by employing 3-D imprinting scaffold fabrication, cell seeding techniques and recent advances in nanotechnology.
The traditional top down strategy in tissue engineering aims at seeding cells in a preformed 3D scaffold of polymers, natural porous materials or decellularized native extracellular matrix. In the bottom up strategy of tissue engineering, various methods like cell printing, microwells, cell sheets and self assembled hydrogels can be used to aggregate cells to form distinct subunits that could then be used as building blocks to engineer whole organs [1].
Currently, two approaches are considered for tooth regeneration:
(a) Scaffold based approach which involves using scaffolds on which cells can be planted either in vitro or by cell homing [Table/Fig-1]. Cell homing compared to cell delivery based tooth regeneration involves in situ induction of endogenous stem cells from adjacent host sites to mobilize and inhabit the native host matrix or implanted scaffold matrix [2]. This method excludes the need for isolation and laboratory manipulation of cells thereby improving clinical success and reducing cost.
Tissue engineering triad: Seeding stem cells onto growth factor laden scaffold matrix in presence of nutrition is used for tissue regeneration
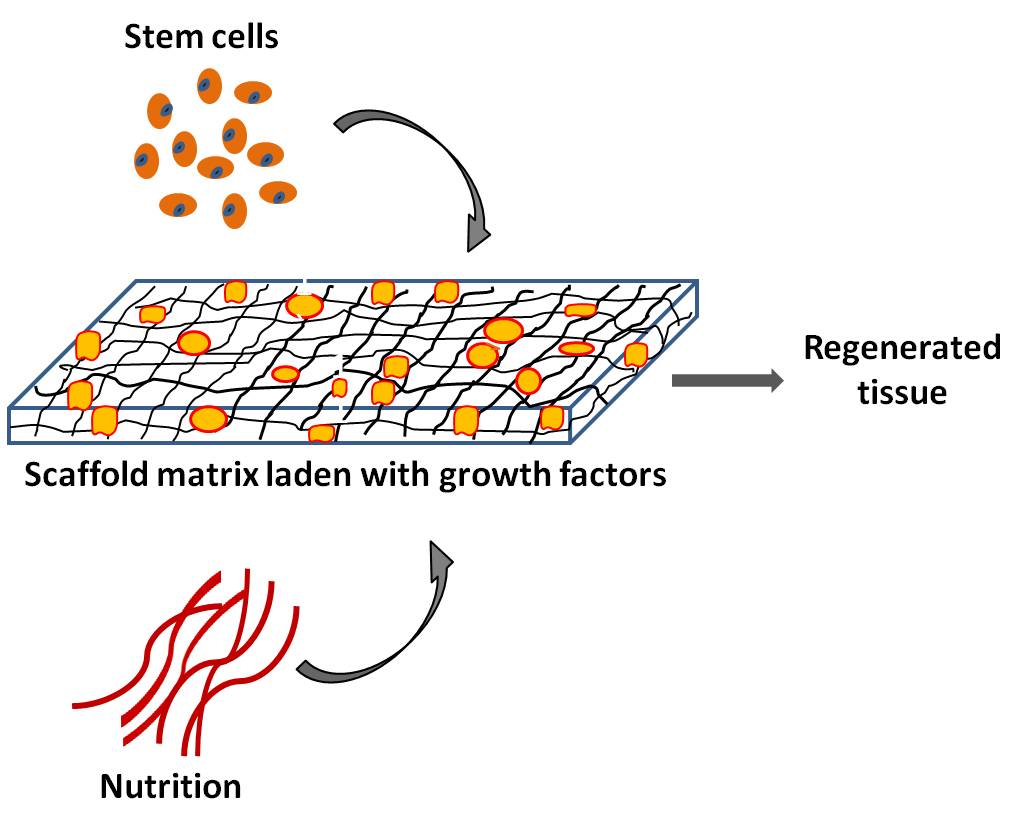
(b) Scaffold free approach aims at directly inducing developmental processes of embryonic tooth formation guided by appropriate signals to produce tooth structures that mimic natural teeth in morphology and size.
Former approach is dependent on use of biomaterials for the fabrication of scaffolds. Determining the physical and mechanical properties of biomaterials and assessing their effect on cell survival and extracellular matrix deposition is imperative for any scaffold based tissue regeneration.
Scaffold Based Tooth Regeneration
Tooth comprises of both hard and soft tissues, hence there may be a need to combine different approaches when regenerating teeth or its individual components. One proposal is to create a three dimensional growth factor rich scaffold construct in the laboratory by computer aided manufacturing system on which cells can be seeded in an organized fashion such that it can produce an exact replica of the tissue with desired size and architecture. Thereafter, maturation of this scaffold can be achieved by its in vivo implantation in animal tissues like omentum or renal capsule so as to receive sufficient blood supply, oxygen and nutrition or in vitro in the laboratory using perfusion or flow based reactors to facilitate diffusion of nutrients and metabolites [3]. Regenerated tissue or organ can then be implanted into the intended recipient site. The challenge however exists to seamlessly integrate the implanted tissue with the pre-existing tissues and placement of rigid scaffolds in sites with complicated morphology without damaging them. Scaffolds with adequate rigidity are recommended when hard tissues are to be generated, so that they can maintain the shape of the future tissue/organ [Table/Fig-2].
Combining (a) stem cells, (b) growth factors and (c) scaffold matrix in a three dimensional tooth construct or injecting into the desired location for regenerating tooth/ individual components
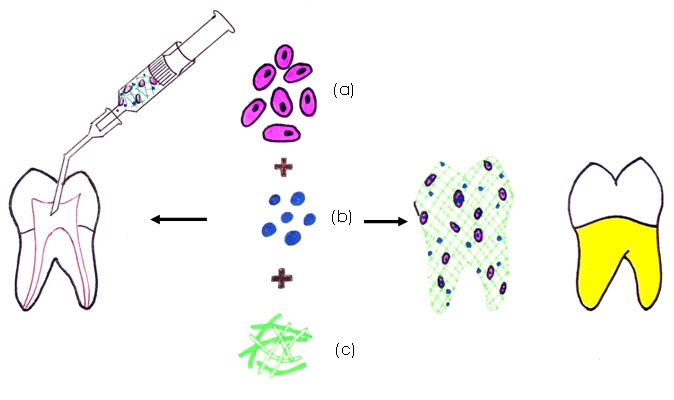
The second proposal involves injecting a soft scaffold matrix impregnated with cells and growth factors into the desired location. This technique is particularly useful in areas of difficult access like inside the pulp cavity which is narrow and tortuous where implanting a rigid matrix would be difficult. Injectable scaffolds are easy to handle and can be delivered by a syringe [Table/Fig-2].
Selection of Biomaterials
To serve as a physical matrix for tooth reconstruction, the scaffold should meet certain general requirements like ease of handling, adequate porosity, biodegradability, bioactivity, good physical and mechanical strengths, low immunogenicity and ability to support vascularity. Adequate pore size, shape and volume are highly desirable in a scaffold to permit penetration and diffusion of cells and/or growth factors as well as nutrients and waste products to and from the cells. Scaffold degradation should match the rate at which new tissue is formed without leaving any noxious byproducts.
Biomaterials for constructing scaffolds can be natural /synthetic and rigid / non rigid. Natural biomaterials offer good cellular compatibility i.e. ability to support cell survival and function thereby enhancing the cells’ performance, and biocompatibility. Their disadvantages include source variability, immunogenicity, if not pure, limited range of mechanical properties and lack of control over pore size. Some natural biomaterials used in tooth regeneration include (a) proteins like collagen, fibrin and silk (b) polysaccharides like chitosan, hyaluronic acid, alginate and agarose.
Unlike natural biomaterials, synthetic biomaterials can be manufactured in unlimited supply under controlled conditions, are cheaper and can be tailored to obtain desired shape, cell differentiation properties and mechanical and chemical properties especially the strength, pore characteristics and degradation rate suited for intended applications. However, synthetic biomaterials lack cell adhesion sites and require chemical modifications to improve cell adhesion. Examples of synthetic biomaterials are organic polymers like poly lactic acid (PLA), poly glycolic acid (PGA), poly lactide-co- glycolic acid (PLGA) and polycaprolactone (PCL). Other synthetic materials include inorganic calcium phosphate materials like hydroxyapatite (HA) or beta tricalcium phosphate (β TCP), and compositions of silicate and phosphate glasses. PLA, PGA, PLGA and PCL are few polymers that are commonly used for forming porous scaffolds.
Rigid biomaterials provide structural substitutes where as soft biomaterials are chosen to provide cell encapsulation. Since a tooth is routinely subjected to mechanical loads, it is crucial that a matrix selected for regenerating hard tissues has adequate strength properties to support the applied loads. Additionally, the regenerated matrix should not undergo any volumetric change lest it induces residual stresses in the tissue predisposing it to fracture. For regenerating pulp within a natural tooth, the matrix selected should preferably be soft and injectable to ease delivery into the complex pulp space, allow cell attachment to the matrix and the surrounding pulp cavity walls, support vascularity and exhibit minimal contraction.
A single matrix may not be an ideal scaffold material. Hybrid scaffolds may be best suited keeping in view the variations in tooth composition. Biomaterials that are promising for tooth regeneration are discussed.
Non Rigid/Soft Biomaterials
Hydrogels are hydrophilic polymers that are suspended in water and serve as soft cell encapsulating scaffolds. They demonstrate sol to gel conversions and hence can be injected, thereby providing convenience of insertion into narrow and tortuous locations. Several methods employed for forming hydrogels include thermal gelation, ionic interaction, physical cross linking, photo polymerization and chemical cross linking [4]. Hydrogel forming polymers that are frequently used in tooth engineering include collagen, fibrin, alginate, hyaluronic acid, poly ethylene glycol and silk.
Collagen
Collagen can be extracted from several allogenic sources as it is the most prevalent structural protein found in the extracellular matrix of various connective tissues like bone, cartilage, tendon, muscle, skin etc. Collagen has been widely tested in dental regenerative studies because of its structural and chemical similarity to the predominant structural protein found in the extracellular matrix of several dental tissues.
It offers advantages of biocompatibility and bioactivity as it naturally promotes cellular adhesion, cellular migration and cell growth. It is degraded by the enzyme, collagenase and degrades with only mild inflammatory reaction. Collagen has a high tensile strength and hence can be woven or twisted into desired form. Its physical strength however is not high but is considered sufficient for pulp regeneration. Cross linking of collagen can modify its mechanical and physical properties. Chemical cross-linking with glutaraldehyde or diphenylphosphoryl azide improves the mechanical stiffness of collagen but at the same time can compromise cell survival and biocompatibility [5]. Forming hybrid scaffolds with β- TCP/polyethylene and HA greatly improves its mechanical properties and enhances bone conductivity [6].
Collagen scaffolds have shown good attachment and growth of DPSCs into the material. The triad of dental pulp stem cells (DPSCs), collagen scaffold, and DMP1 has shown to induce formation of an organized matrix similar to that of pulpal tissue capable of hard tissue formation [7]. In one study, osteodentin was seen to be formed only with Type I collagen scaffold suggesting certain physicochemical surface characteristics required for odontoblast differentiation [8]. The RGD site in fibronectin is believed to be critical for cell attachment.
In another study, collagen scaffolds laden with series of growth factors like basic fibroblast growth factor (bFGF), vascular endothelial growth factor (VEGF) and/or platelet derived growth factor (PDGF) were implanted into endodontically treated root canals. In vivo implantation of these endodontically treated real-sized native human teeth in mouse dorsum for 3 weeks revealed re-cellularized and revascularized dental pulp tissue that integrated to native dentinal wall in root canals with neo dentin formation [2].
Collagen sponges for regeneration in bone defects and collagen membranes in guided bone and tissue regeneration are currently being used extensively in clinical conditions. Pulp cells, however, are also known to cause significant contraction of collagen [9]. This drawback needs to be addressed when using collagen scaffolds for regenerating pulp.
Fibrin
Fibrin is a natural biomaterial produced by polymerizing the protein fibrinogen present in the plasma under the enzymatic activity of thrombin. It is advantageous compared to synthetic polymers and collagen gels when cost, biocompatibility, immune response and cell adhesion properties are concerned. It can be obtained from autologous sources hence has no undesirable immunogenic reactions in addition to being reproducible several times. Fibrin however undergoes rapid shrinkage, degradation and has low mechanical stiffness. It’s shrinkage can be reduced by fixing agents like poly L lysine where as degradation rate can be controlled by fibril cross-linking or by use of enzyme inhibitors [10]. Mechanical properties are seen to greatly improve when fibrin is used as a composite scaffold with polyurethane, β-TCP or polyethylene and hyaluronic acid [11]. Physical characteristics, cell invasion and survival characteristics of fibrin scaffold can be adjusted by manipulating the concentration of fibrinogen/thrombin components. Increasing fibrinogen content enhances stiffness but at the same time reduces porosity [12].
Fibroblasts have shown to produce collagen in response to transforming growth factor beta (TGF-β) in a fibrin scaffold, actively reorganize fibrin matrix and subsequently remodel it into a collagen containing scar like tissue [13]. Fibrin matrix provides a suitable environment for angiogenesis as well. Addition of pro angiogenic growth factors and their controlled release results in forming functional well organized structures [14]. Fibrin hydrogels are injectable and additionally can be molded into 3-D shapes. All these features render fibrin a suitable scaffold material for pulp regeneration.
Platelet-rich fibrin (PRF)/ platelet rich plasma (PRP) is a component of blood in which platelets are concentrated and is rich in cytokines and growth factors especially PDGF and TGF-β. PDGF binds to endothelial cells to initiate capillary in growth where as TGF-β binds to osteoblasts and stem cells to initiate mitosis and stimulate bone formation. When added into fibrin glue, PRP/PRF enriches the microenvironment with growth factors. Dental bud cells suspended into fibrin glue and enclosed with PRF when transplanted in porcine model have shown to regenerate a complete tooth with crown, root, pulp, enamel, dentin, cementum, blood vessels, and periodontal ligament in indiscriminate shape [15]. PRP/ PRF along with collagen membrane currently is being extensively used for regeneration of periodontal ligament and alveolar bone as a part of guided tissue regeneration procedures in periodontics and oral surgery.
Alginate
Alginate is a natural polysaccharide that offers benefits of biocompatibility and non toxicity but exhibits low mechanical stiffness and uncontrolled in vivo degradation rate. Its mechanical strength can be improved by increasing calcium content and cross linking density. Stable covalent cross linking compared to ionic cross linking offers higher mechanical strengths in alginate hydrogels [16]. Arginine-glycine-aspartic acid (RGD) modified alginate hydrogel promotes cell adhesion, spreading, proliferation and differentiation.
Alginate hydrogels provide an appropriate matrix on which dentin pulp regeneration and periodontal regeneration can take place and may also be useful for delivery of growth factors like TGF β, to enhance the natural regenerative capacity of the dental pulp. Both TGF β containing and acid treated alginate hydrogels up regulate dentin matrix secretion and induce odontoblast like cell differentiation with secretion of regular tubular dentin matrix [17]. Human periodontal ligament fibroblast cells (hPDLF) adhere and proliferate well on alginate/bioglass composite scaffolds compared to alginate only scaffolds. The presence of nano bioactive glass ceramic (nBGC) enhances the alkaline phosphatase (ALP) activity of the hPDLF cells cultured on these composite scaffolds [18].
Hyaluronic Acid
Hyaluronic acid, one of the glycosaminoglycans present in the extracellular matrix of connective tissue, offers excellent potential as scaffold for tissue regeneration. It is biocompatible and has low immunogenic potential but poor mechanical strength and rapid in vivo degradation rate which can however be controlled by cross linking and chemical modification of the polymers [19]. It is enzymatically degraded by hyaluronidase to nontoxic products that are easily processed by the body. A composite of alginate and hyaluronic acid gel has shown improved physical, mechanical and biological properties [20]. RGD peptides in hyaluronic acid hydrogel enhance cellular attachment, cellular spreading and proliferation [21].
Hyaluronic acid gels are injectable and hence serve as suitable scaffolds for pulp regeneration. Hyaluronic acid and collagen sponges have shown to induce and sustain dental pulp proliferation and invasion of vessels from amputated dental pulp. Hyaluronic acid sponge induces less inflammatory response compared to collagen sponge [22].
Poly (Ethylene Glycol)
Poly (ethylene glycol) (PEG) is a synthetic polymer and offers advantages of non toxicity, biocompatibility, low immunogenicity and ability to undergo in vivo degradation. Its resistance to cellular and protein adsorption reduces recognition by immune system and therefore its rejection. In its hydrogel form, it has been widely tested as a scaffold material for tissue regeneration. PEG hydrogels modified with cell adhesion RGD peptides improves cell adhesion, cell survival and matrix synthesis within the 3 D scaffold network [23]. The mechanical strength of PEG hydrogel is determined by the molecular weight, cross linking and concentration of polymers. Its elastic modulus can be increased by decreasing the molecular weight or increasing the concentration of the polymer [24]. Producing hydrogel by photopolymerization is preferable over thermal polymerization as it is more convenient, efficient, rapid and less fragile towards encapsulated proteins and cells [25].
Incorporation of hydrolytically degradable poly (lactic acid) (PLA) groups greatly increase the potential applications of PEG hydrogels. Tri block copolymers of PLA-PEG-PLA, containing PLA blocks and acrylate end groups have been used to create photopolymerizable and hydrolytically degradable hydrogels for controlled release of bio active molecules [26]. The degradation rate of PLA-PEG-PLA hydrogels can be pre engineered by changing the polymer content of the hydrogels or length of the degradable PLA segments [27].
PEGylated fibrin hydrogel has shown to serve as a suitable scaffold for growth of DPSCs and PDLSCs wherein porous PEG hydrogel provided mechanical and structural support and fibrin induced formation of vascularized tissue [28].
Chitosan
Chitosan is a derivative of chitin, a natural biopolymer which is biocompatible, biodegradable, antimicrobial and possesses tissue healing and osteoinductive effects. It has the ability to bind to growth factors, glycosaminoglycans and DNA and can be easily processed into membranes, gels, nanofibres, beads, scaffolds and sponges. Because of these properties, chitosan gel alone or in combination with demineralized bone matrix/collagenous membrane is quite promising in periodontal regeneration [29]. HA chitosan scaffolds are effective biomaterials for bone tissue regeneration [30].
Silk
Silk scaffolds offer biocompatibility, non toxicity, diverse physical characteristics and ability towards cell attachment and proliferation. Silk gel material is able to create a lasting three dimensional soft tissue augmentation hence is useful in periodontal and maxillofacial therapies. Hexafluoroisopropanol (HFIP) based silk is slower in degradation and supports soft dental pulp formation better than aqueous based silk. Tooth bud cells seeded on HFIP silk scaffolds, with or without incorporated RGD peptides have shown to form mineralized tissue indicating the usefulness of these scaffolds in osteodentin formation [31]. Recently, micron sized silk fibres (10-600μm) have been incorporated as a reinforcement in compact fibre composite to produce high strength biomaterial that would serve as load bearing bone grafts. These have favored hBMSC differentiation and formation of bone like tissue suggesting their use for bone engineering applications [32].
Rigid Biomaterials
Polylactic Acid (PLA), Polyglycolic Acid (PGA), Polylactide-Co-Glycolide (PLGA)
These are synthetic polyester polymers that are biocompatible, biodegradable and mild inflammatory. Their structure, viscosity, porosity, degradation rate and release of incorporated bio molecules can be adjusted by factors like polymer ratio, molecular weight and crystallinity thereby offering great design flexibilities. PLA and PGA on degradation produce lactic acid and glycolic acid respectively which are naturally removed through metabolic pathways and excreted through urine. PLGA is a copolymer with desirable physical and mechanical properties including their degradation rate that can be adjusted by regulating the lactic and glycolic portion of the copolymer.
Both PLA and PGA scaffolds have shown to be conducive for seeding of stem cells like SHED, DPSCs and dental pulp fibroblasts. Stem cells have shown differentiation into odontoblast like cells and endothelial cells on these scaffolds and ability to generate tissues similar to dental pulp and dentin. PGA has been found to be a more conducive scaffold for dental pulp cell proliferation than hydrogel and alginate [33]. The shape of scaffold matrix: porous, powdered or fiber mesh has also shown to have an effect on the type of tissue generated [34]. PLGA hydrogels combined with PEG or recombinant human growth/ differentiation factor 5 ( rhGDF-5) offers advantages of injectability, minimal immune response, controlled release of bio molecules, gene delivery and bone maturation with lamellar bone formation [35].
Ceramic Scaffolds
This group of scaffolds refers to calcium/phosphate materials, bioactive glasses and glass ceramics. These have long been used in healing of large bone defects. Ca/P scaffolds include β-TCP or HA and have been widely tested for bone regeneration owing to their properties of resorption, biocompatibility, low immunogenicity, osteoconductivity, bone bonding and similarity to mineralized tissues. 3D CaP porous granules have proved useful in dental tissue engineering by providing favourable 3D substrate conditions for hDPSC growth and odontogenic differentiation [36]. Addition of SiO2 and ZnO dopants to pure TCP scaffolds increases its mechanical strength as well as cellular proliferation properties [37].
Glass ceramics based on SiO2-Na2O-CaO-P2O5 are bioactive and offer good crystallization conditions. Release of dissolution products such as calcium phosphate enhances the osteoblastic activity of the material. Certain disadvantages associated with their use are difficulty of shaping, poor mechanical strength, brittleness, slow degradation rate and high density which have restricted their use as scaffolds in bone tissue regeneration.
Scaffolds made of ceramic can be modified to obtain desired permeability, controlled dissolution rate and specific surface characteristics to enhance cellular activity. Change in pore size and volume affects the mechanical stiffness of the scaffold [38]. Mg based glass ceramics have improved mechanical integrity and high rate of bioactivity [39]. Niobium doped fluorapatite glass ceramic displays excellent attachment, proliferation and differentiation of hDPSCs on its surface [40].
Composite Scaffold
A composite scaffold made up of two different scaffold materials like synthetic polymer and inorganic materials combines the advantages of each individual material and minimizes their disadvantages. Polymer materials lack adequate stiffness. Addition of stiff materials like glasses and ceramic overcomes the inherent weakness of polymers making it suitable for dental tissue regeneration. Moreover, polymers have the added advantage of ease of fabrication compared to ceramic scaffolds which are brittle and have poor processability to form highly porous structures.Also, resorption products of Ca/P scaffolds are expected to neutralize the acidic pH created by the acidic degradation products of polymers thereby reducing the mild inflammation associated with the degradation of polymers.
Highly porous polymer/ceramic composite scaffold appears to be a promising substrate for bone tissue engineering due to its excellent mechanical properties and osteoconductivity. Blaker et al., 2003 [41] indicated improved cell adhesion, spreading and viability of cells grown on polymer-bioglass-composites and also confirmed the high bioactivity and biocompatibility of the material for hard tissue repair. Pure PLGA scaffold and three composite scaffolds: PLGA/HA, PLGA/TCP and PLGA/CDHA when studied for DPSC proliferation and differentiation suggested that Ca/P composite scaffolds effectively supported regeneration of tooth tissue. Amongst the four scaffolds, PLGA/TCP scaffold was most suitable for dentin pulp regeneration [42].
A porous zirconia hydroxyapatite (ZrO(2)/HA) composite scaffold has excellent mechanical properties with cellular/tissue compatibility and can be a promising substrate to achieve both bone reconstruction and regeneration needed in the treatment of large bone defects [43].
Polycaprolactone (PCL)
PCL is a synthetic polyester polymer with limited bioactivity and an extended degradation rate. It’s degradation rate can be modified by changing molecular weight, crystallinity or by modifying the structure of PCL with Hydrophilic polyethylene glycol, ceramics or making copolymers with PLA or PGA [44]. Surface and mechanical properties of PCL can be modified by coating with HA, TCP, gelatin and calcium phosphate, bone sialoprotein or collagen to promote osteoblast and endothelial cell adhesion, migration and proliferation [45,46].
Composite scaffolds of PCL and PGA seeded with genetically modified human cells have shown generation of cementum like tissue, ligament and bone structures suggesting their potential in periodontal regeneration [47].
Use of Nanodelivery in Designing Scaffolds
Introduction of nano sized particles has tremendously revolutionized tissue engineering. At significantly smaller sizes, these particles greatly increase the surface area per unit volume and the quatum effects compared to large sized particles, thereby improving the performance behavior of materials [48]. Collective advancements in nanotechnology have enabled the fabrication of innovative scaffolds like composite nanofibrous scaffolds that simulate the matrix environment in which cells can be accommodated to proliferate and differentiate towards desired lineages [Table/Fig-3] [49]. Nano meter sized fibers can be processed to form highly porous scaffolds that will allow easy cell migration and nutrient diffusion. Not only do they provide a large surface area for cell attachment but also because of increased porosity permit the delivery of drugs and growth factors, thereby allowing interaction between cells and extracellular matrix as seen during normal tissue development.
Use of nanotechnology for fabrication of innovative scaffolds in dental tissue engineering. Scanning electron microscopy shows (A) Porous PLGA sponge fabricated using salt-leaching techniques. (B) PLGA microspheres of differential sizes encapsulating growth factors and having smooth spherical surface. (C) PLGA nanofibers fabricated using electrospining techniques. Phase contrast image shows (D) PLGA microspheres in chitosan-based gels for advanced controlled delivery and cell interaction. (Reprinted from Ref. [49] with permission from Elsevier Copyright 2007)
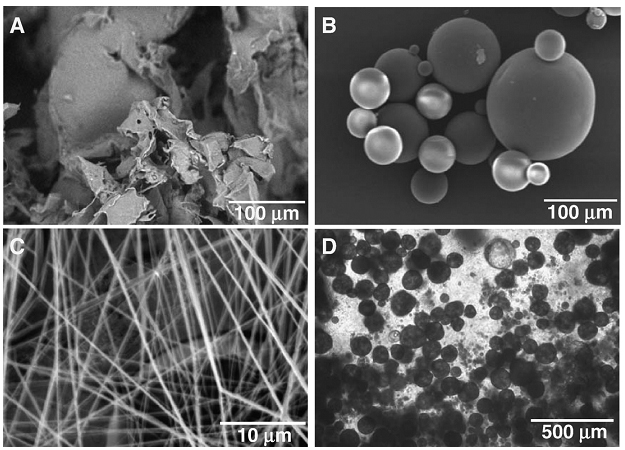
Nano fibrous scaffolds that will promote functional tissue regeneration can be created by simple coating methods, blending of polymeric- bioactive molecules or by surface modification methods. Hydrophilization, electrospinning and more recently electrospin-electrospraying are few methods that enable the synthesis of polymeric and composite nano scaffolds suited for specific biomedical applications [50].
Creating a controlled microtexture on implant surfaces via additive surface modification techniques with bioactive nanohydroxyapatite have shown to positively influence guided tissue regeneration. Nanostructured HA compared to bulk HA provides close contact with surrounding tissues and quick resorption characteristics. It has also shown to increase the proliferation rate of PDL cells possibly by activating the epidermal growth factor and its downstream targets [51]. Modifying the polymer surface with cell adhesive ligands like arginine-glycine-aspartic acid (RGD) peptide derived from fibronectin improves cell adhesion and interaction. Huang et al., 2008 [52] studied the effect of artificial bioactive nano structures– branched peptide amphiphile molecules containing RGD (BRGD-PA) on ameloblasts like cells and enamel organ epithelial cells of mouse embryonic incisors. In both cell and organ culture models, enhanced cell attachment and proliferation with greater enamel specific protein expression levels were seen. Introducing cell cleavable groups in polymeric hydrogels also improved cell penetration.
Large complex 3D scaffolds laden with microvascular networks and growth factor concentration gradients have recently been designed using microfluidics to promote culturing of multiple cell types and guide cell growth in a controlled space [Table/Fig-4] [53]. Encapsulated cells within 200 μm of the microfluidic channels have shown the best survival, suggesting that microchannels provide conduits for diffusion of nutrients and metabolites to seeded cells thereby enhancing neovascularization and/or cell metabolism which is especially important when regenerating dental pulp [53].
Fabrication of microfluidic devices, including (a) the standard soft-lithography-based fabrication process, (b) the design, analysis, and optimization of the device, (c) the creation of the master molds, (d) the fabrication of scaffolds from the masters, and (e) integration into a benchtop system (f) Demonstration of approaches developed for advanced organ mimetic microfluidic devices. (Reprinted from Ref. [53] with permission from Elsevier Copyright 2011)
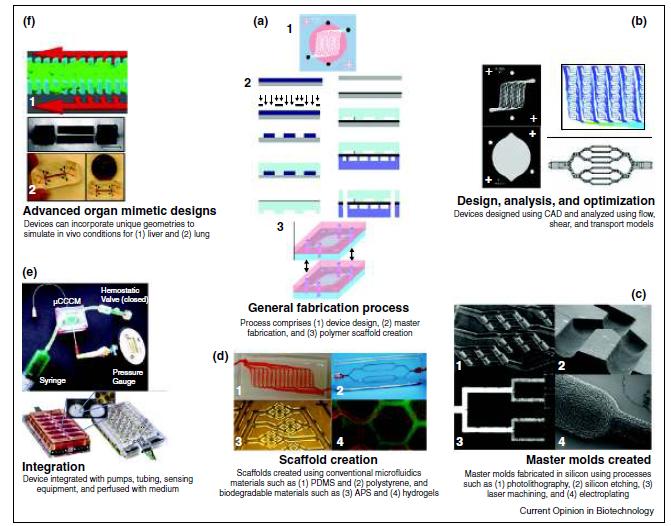
Nano Particles for Drug Delivery
Incorporating bioactive molecules in matrix materials enhances varying regenerative capacities. Nanotechnology has made it possible to deliver growth factors into the scaffold matrix by use of vehicles like agarose beads, collagen sponge, alginate gels, sandwich collagen membrane, hydrogel microspheres and β TCP and release them locally into the scaffold at appropriate times.
Microspheres are drug encapsulating polymer matrices used for slow and prolonged release of drugs [Table/Fig-3] [49]. Low molecular weight polymers form porous microspheres that release drug rapidly while high molecular weight polymers form dense microspheres that release drug slowly. PLGA microspheres that release vascular endothelial growth factor (VEGF) when delivered into porous scaffolds have provided prolonged release of growth factor for up to 21 days, resulting in significantly enhanced angiogenesis [54]. Nanotubes compared to spheres for drug delivery provide larger inner volumes for filling desired chemical or biochemical species and offers distinct inner and outer surfaces that can be differentially functionalized [55]. Complex drug delivery systems that will render differential release of multiple bioactive molecules in regulated and sustained doses and provide multiple signaling requirements need to further researched.
Conclusion
Tissue engineering is one of the latest emerging innovations and its potential is being vastly experimented and utilized in various branches of medicine. Biomaterials are indispensable in tissue regeneration as they serve as porous frameworks over which all other ingredients for tissue regeneration are laid. Research related to biomaterials has mainly focused towards constructing scaffolds with optimum physical, chemical and mechanical properties while including biomolecules and their controlled delivery to promote cell survival and cell-scaffold interactions. With the advent of micro scale and nano scale technologies, it has become possible to design and fabricate scaffolds that are not only large three dimensional constructs but also provide a three dimensional micro environment that supports cell behavior, tissue function, implantation and host integration. Though, tremendous research still needs to be done to regenerate tissues with clinically applicable structure and function; nevertheless, the applicability of stem cells and biomaterial sciences for dental reconstruction cannot be understated and offers a promising future.
[1]. Horst OV, Chavez MG, Jheon AH, Desai T, Klein OD, Stem cell and biomaterials research in dental tissue engineering and regeneration Dent Clin North Am 2012 56(3):495-520. [Google Scholar]
[2]. Kim JY, Xin X, Moioli EK, Chung J, Lee CH, Chen M, Fu SY, Koch PD, Mao JJ, Regeneration of dental-pulp-like tissue by chemotaxis-induced cell homing Tissue Eng Part A 2010 16:3023-31. [Google Scholar]
[3]. Duailibi SE, Duailibi MT, Zhang W, Asrican R, Vacanti JP, Yelick PC, Bioengineered dental tissues grown in the rat jaw J Dent Res 2008 87:745-50. [Google Scholar]
[4]. Tan H, Marra KG, Injectable, biodegradable hydrogels for tissue engineering applications Materials 2010 3:1746-67. [Google Scholar]
[5]. Wu X, Black L, Santacana-Laffitte G, Patrick CW Jr, Preparation and assessment of glutaraldehyde-crosslinked collagen-chitosan gels for adipose tissue engineering J Biomed Mater Res A 2007 81:59-65. [Google Scholar]
[6]. Wahl DA, Sachlos E, Liu C, Czernuszka JT, Controlling the processing of collagen-hydroxyapatite scaffolds for bone tissue engineering J Mater Sci Mater Med 2007 18:201-9. [Google Scholar]
[7]. Prescott RS, Alsanea R, Fayad MI, Johnson BR, Wenckus CS, Hao J, John AS, George A, In vivo generation of dental pulp - like tissue by using dental pulp stem cells, a collagen scaffold, and dentin matrix protein 1 after subcutaneous transplantation in mice J Endod 2008 34:421-26. [Google Scholar]
[8]. Nakashima M, Induction of dentin in amputated pulp of the dogs by recombinant human bone morphogenic proteins-2 and -4 with collagen matrix Archs Oral Biol 1994 39:1085-9. [Google Scholar]
[9]. Chan C, Lan W, Chang M, Chen Y, Lan W, Chang H, Effects of TGF - betas on the growth, collagen synthesis and collagen lattice contraction of human dental pulp fibroblasts in vitro Arch Oral Biol 2005 50:469-79. [Google Scholar]
[10]. Jockenhoevel S, Zund G, Hoerstrup SP, Chalabi K, Sachweh JS, Demircan L, Messmer BJ, Turina M, Fibrin gel-advantages of a new scaffold in cardiovascular tissue engineering Eur J Cardiothorac Surg 2001 19:424-30. [Google Scholar]
[11]. Lee F, Kurisawa M, Formation and stability of interpenetrating polymer network hydrogels consisting of fibrin and hyaluronic acid for tissue engineering Acta Biomater 2013 9:5143-52. [Google Scholar]
[12]. Linnes MP, Ratner BD, Giachelli CM, A fibrinogen based precision microporous scaffold for tissue engineering Biomaterials 2007 28:5298-306. [Google Scholar]
[13]. Clark RA, Nielsen LD, Welch MP, McPherson JM, Collagen matrices attenuate the collagen-synthetic response of cultured fibroblasts to TGF-beta J Cell Sci 1995 108(Pt 3):1251-61. [Google Scholar]
[14]. Ehrbar M, Djonov VG, Schnell C, Tschanz SA, Martiny-Baron G, Schenk U, Wood J, Burri PH, Hubbell JA, Zisch AH, Cell-demanded liberation of VEGF121 from fibrin implants induces local and controlled blood vessel growth Circ Res 2004 94:1124-32. [Google Scholar]
[15]. Yang KC, Wang CH, Chang HH, Chan WP, Chi CH, Kuo TF, Fibrin glue mixed with platelet-rich fibrin as a scaffold seeded with dental bud cells for tooth regeneration J Tissue Eng Regen Med 2012 6:777-85. [Google Scholar]
[16]. Sakai S, Kawakami K, Synthesis and characterization of both ionically and enzymatically cross-linkable alginate Acta Biomater 2007 3:495-501. [Google Scholar]
[17]. Dobie K, Smith G, Sloan AJ, Smith AJ, Effects of alginate hydrogels and TGF-beta 1 on human dental pulp repair in vitro Connect Tissue Res 2002 43:387-90. [Google Scholar]
[18]. Srinivasan S, Jayasree R, Chennazhi KP, Nair SV, Jayakumar R, Biocompatible alginate/nano bioactive glass ceramic composite scaffolds for periodontal tissue regeneration Carbohydrate Polymers 2012 87:274-83. [Google Scholar]
[19]. Ouasti S, Donno R, Cellesi F, Sherratt MJ, Terenghi G, Tirelli N, Network connectivity, mechanical properties and cell adhesion for hyaluronic acid/PEG hydrogels Biomaterials 2011 32:6456-70. [Google Scholar]
[20]. Ganesh N, Hanna C, Nair SV, Nair LS, Enzymatically cross-linked alginic-hyaluronic acid composite hydrogels as cell delivery vehicles Int J Biol Macromol 2013 55:289-94. [Google Scholar]
[21]. Park YD, Tirelli N, Hubbell JA, Photopolymerized hyaluronic acid based hydrogels and interpenetrating networks Biomaterials 2003 24:893-900. [Google Scholar]
[22]. Inuyama Y, Kitamura C, Nishihara T, Morotomi T, Nagayoshi M, Tabata Y, Matsuo K, Chen KK, Terashita M, Effects of hyaluronic acid sponge as a scaffold on odotoblastic cell line and amputated dental pulp J Biomed Mater Res B. Appl Biomater 2010 92:120-8. [Google Scholar]
[23]. Burdick J, Ansetn K, Photoencapsulation of osteoblasts in injectable RGD-modified PEG hydrogels for bone tissue engineering Biomaterials 2002 23:4315-23. [Google Scholar]
[24]. Zhu J, Bioactive modification of poly(ethylene glycol) hydrogels for tissue engineering Biomaterials 2010 31:4639-56. [Google Scholar]
[25]. Papadopoulos A, Bichara DA, Zhao X, Ibusuki S, Randolph MA, Anseth KS, Yaremchuk MJ, Injectable and photopolymerizable tissue engineered auricular cartilage using PEGDM copolymer hydrogels Tissue Eng Part A 2011 17:161-9. [Google Scholar]
[26]. Mason MN, Metters AT, Bowman CN, Anseth KS, Predicting controlled-release behavior of degradable PLA-b-PEG-b-PLA hydrogels Macromolecules 2001 34:4630-35. [Google Scholar]
[27]. Al Nasassrah MA, Podczeck F, Newton JM, The effect of an increase in chain length on the mechanical properties of polyethylene glycols Eur J Pharm Biopharm 1998 46:31-38. [Google Scholar]
[28]. Jiang B, Waller TM, Larson JC, Appel AA, Brey EM, Fibrin-loaded porous poly(ethylene glycol) hydrogels as scaffold materials for vascularized tissue formation Tissue Eng Part A 2013 19:224-34. [Google Scholar]
[29]. Boynuegri D, Ozcan G, Senel S, Uç D, Uraz A, Ogüs E, Cakilci B, Karaduman B, Clinical and radiographic evaluations of chitosan gel in periodontal intraosseous defects: a pilot study J Biomed Mater Res B Appl Biomater 2009 90:461-6. [Google Scholar]
[30]. Tanase CE, Sartoris A, Popa MI, Verestiuc L, Unger RE, Kirkpatrick CJ, In vitro evaluation of biomimetic chitosan-calcium phosphate scaffolds with potential application in bone tissue engineering Biomed Mater 2013 8:025002doi: 10.1088/1748-6041/8/2/025002 [Google Scholar]
[31]. Zhang W, Ahluwalia IP, Literman R, Kaplan DL, Yelick PC, Human dental pulp progenitor cell behavior on aqueous and hexafluoroisopropanol (HFIP) based silk scaffolds J Biomed Mater Res A 2011 97:414-22. [Google Scholar]
[32]. Mandal BB, Grinberg A, Gil ES, Panilaitis B, Kaplan DL, High-strength silk protein scaffolds for bone repair PNAS 2012 109:7699-704. [Google Scholar]
[33]. Bohl K, Shon J, Rutherford B, Mooney DJ, Role of synthetic extracellular matrix in development of engineered dental pulp J of Biomater Sci. Polymer Ed 1998 9:749-64. [Google Scholar]
[34]. Tonomura A, Mizuno D, Hisada A, Kuno N, Ando Y, Sumita Y, Honda MJ, Satomura K, Sakurai H, Ueda M, Kagami H, Differential effect of scaffold shape on dentin regeneration Ann Biomed Eng 2010 38:664-71. [Google Scholar]
[35]. Jeong JH, Kim SW, Park TG, Biodegradable triblock copolymer of PLGA-PEG-PLGA enhances gene transfection efficiency Pharm Res 2004 21:50-4. [Google Scholar]
[36]. Nam S, Won JE, Kim CH, Kim HW, Odontogenic differentiation of human dental pulp stem cells stimulated by the calcium phosphate porous granules J Tissue. Eng Vol 2011, Article ID 812547, 10 pages, doi:10.4061/2011/812547 [Google Scholar]
[37]. Fielding GA, Bandyopadhyay A, Bose S, Effects of silica and zinc oxide doping on mechanical and biological properties of 3D printed tricalcium phosphate tissue engineering scaffolds Dent Mater 2012 28:113-22. [Google Scholar]
[38]. Li SH, de Wijn JR, Li JP, Layrolle P, de Groot KD, Macroporous biphasic calcium phosphate scaffold with high permeability/porosity ratio Tissue Engineering 2003 9:535-48. [Google Scholar]
[39]. Goudouri OM, Theodosoglou E, Theocharidou A, Kontonasaki E, Papadopoulou L, Chatzistavrou X, Koidis P, Paraskevopoulos KM, Magnesium based sol-gel derived bioactive glass ceramics for dental tissue regeneration Key Engineering Materials 2012 493/494:884-89. [Google Scholar]
[40]. Kushwaha M, Pan X, Holloway JA, Denry IL, Differentiation of human mesenchymal stem cells on niobium-doped fluorapatite glass–ceramics Dental Materials 2012 28:252-60. [Google Scholar]
[41]. Blaker JJ, Gough JE, Maquet V, Notingher I, Boccaccini AR, In vitro evaluation of novel bioactive composites based on bioglass-filled polylactide foams for bone tissue engineering scaffolds J Biomed Mater Res A 2003 67:1401-11. [Google Scholar]
[42]. Zheng L, Yang F, Shen H, Hua X, Mochizuki C, Sato M, Wang S, Zhang Y, The effect of composition of calcium phosphate composite scaffolds on the formation of tooth tissue from human dental pulp stem cells Biomaterials 2011 32:7053-59. [Google Scholar]
[43]. An SH, Matsumoto T, Miyajima H, Nakahira A, Kim KH, Imazato S, Porous zirconia/hydroxyapatite scaffolds for bone reconstruction Dent Mater 2012 28:1221-31. [Google Scholar]
[44]. Huang MH, Li S, Hutmacher DW, Schantz JT, Vacanti CA, Braud C, Vert M, Degradation and cell culture studies on block copolymers prepared by ring opening polymerization of β-caprolactone in the presence of poly (ethylene glycol) J Biomed Mater Res A 2004 69:417-27. [Google Scholar]
[45]. Erisken C, Kalyon DM, Wang H, Functionally Graded electrospun polycaprolactone and beta-tricalcium phosphate nanocomposites for tissue engineering applications Biomaterials 2008 29:4065-73. [Google Scholar]
[46]. Venugopal J, Zhang YZ, Ramakrishna S, Fabrication of modified and functionalized polycaprolactone nanofibre scaffolds for vascular tissue engineering Nanotechnology 2005 16:2138-42. [Google Scholar]
[47]. Park CH, Rios HF, Jin Q, Bland ME, Flanagan CL, Hollister SJ, Giannobile WV, Biomimetic hybrid scaffolds for engineering human tooth-ligament interfaces Biomaterials 2010 31:5945-52. [Google Scholar]
[48]. Lee SJ, Atala A, Scaffold technologies for controlling cell behavior in tissue engineering Biomed Mater 2013 8(1):010201doi: 10. 1088/1748-6041/8/1/010201 [Google Scholar]
[49]. Moioli EK, Clark PA, Xin X, Lal S, Mao JJ, Matrices and scaffolds for drug delivery in dental, oral and craniofacial tissue engineering Advanced Drug Delivery Reviews 2007 59:308-324. [Google Scholar]
[50]. Rim NG, Shin CS, Shin H, Current approaches to electrospun nanofibers for tissue engineering Biomed Mater 2013 8(1):014102doi: 10.1088/1748-6041/8/1/014102 [Google Scholar]
[51]. Kasaj A, Willershausen B, Reichert C, Rohrig B, Smeets R, Schmidt M, Ability of nanocrystalline hydroxyapatite to promote human periodontal ligament cell proliferation Journal of oral sciences 2008 50:279-85. [Google Scholar]
[52]. Huang Z, Sargeant TD, Hulvat JF, Mata A, Bringas P Jr, Koh CY, Stupp SI, Snead ML, Bioactive nanofibers instruct cells to proliferate and differentiate during enamel regeneration J Bone Miner Res 2008 23:1995-2006. [Google Scholar]
[53]. Inamdar NK, Borenstein JT, Microfluidic cell culture models for tissue engineering Current Opinion in Biotechnology 2011 22:681-9. [Google Scholar]
[54]. Ennett AB, Kaigler D, Mooney DJ, Temporally regulated delivery of VEGF in vitro and in vivo J Biomed Mater Res A 2006 79:176-84. [Google Scholar]
[55]. Kohli P, Martin C, Smart nanotubes for biomedical and biotechnological applications Drug News Perspect 2003 16:566-73. [Google Scholar]