Introduction
The body have an inherent capacity to heal and regenerate tissue damage. However, regeneration is limited and a number of factors are involved (e.g., tissue type, or growth hormones). Any major tissue injury or damage beyond a certain size needs external support or organ transplantation. With increasing number of ageing population and the dangers of increasing number of accidental trauma or diseases leading to the loss in organs or tissues, availability of organ or tissue donors plays an important role. Though science had made a significant progress towards addressing this gap by making autograft and allografts of tissues a possibility [1], but shortage of donors is something which still poses a serious challenge. Every year number of patients across the globe die waiting for a suitable donor [2]. This is because the number of people on the waiting list (recipients) continues to be much larger than the number of both, donors and transplants [3]. As per 2017 data from UNOS and U.S. Government Information on Organ Donation and Transplantation, organ donation is required only in 0.3% deaths, and almost 20 people die each day waiting for organ transplant [4]. Even if one finds a suitable donor, high transplant costs and immunogenic rejections still remain two major hurdles for the recipients.
Three-dimensional (3D) printing is also called Additive Manufacturing (AM). It is a process through which a three-dimensional solid model of any shape can be generated using digital input. The science of medical 3D printing has been a pipeline dream for many years. The first 3D printing patent was filed by Hull CW in 1980s [5]. Hull CW came up with the idea of applying successive layers of base material on top of each other to create a 3D print of any object [5]. Since then, not only medical, but 3D printing has impacted several fields including engineering, manufacturing, and medicine.
Though initially recognised as a buzzword, the concept of tissue regeneration has been popularised these days and has led to terms like Tissue Engineering (TE) or Regenerative Medicine (RM). Tissue engineering is a concept which utilises the principles of biological and engineering techniques to provide damaged tissue replacements [6]. The scaffolds produced by 3D bioprinting approach have been made in order to support cell interaction, and strong enough to provide physical support to freshly created tissue [7]. Introducing stem cells in 3D bioprinted scaffolds had been found to offer great potential for creating replacement tissues [8].
In general, 3D printing process uses powder, plastics, metals, liquid or even cells. These materials can be fused or deposited into or on a substrate, thus making the process highly adaptable to the requirement. The process is accurate, cost-effective and reproducible, such that it can reliably support the production of custom tissues. This also provides an opportunity between clinicians, engineers and researchers to collaborate in order to produce a physical object from a digital file, which was transmitted and downloaded through internet.
Therefore, 3D bioprinting plays an important role in medical field for fabrication of various human and animal tissues and organs, surgical implants and anatomical models for diagnosis and education. The aim of this paper is to present an overview of medical application of 3D printing techniques. Current trends in research and their implications in medical field has also been presented.
Three-Dimensional (3D) Bioprinting
Five technical steps are required to finalise a printed model. The first step involves identifying the anatomy of the target area. Then the 3D geometry is developed through processing of structural inputs in the form of Computed Tomography (CT) scan or Magnetic Resonance Imaging (MRI). The file thus created is optimised for physical printing. Then appropriate 3D printer and materials are carefully selected. The file is further processed to create layered slices of the model. These sections are then fed to the printer for printing. The printing starts from the base layer and subsequently a series of layers are printed (or built) on top until the entire model is built. A schematic diagram of 3D bioprinting process is presented in [Table/Fig-1].
Overview of organ 3D bioprinting workflow.
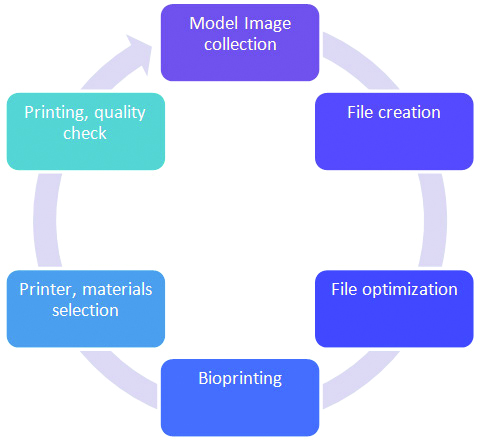
The first step of 3D bioprinting involves identifying the target area anatomy. The 3D geometry file is created, and later optimised for physical printing. Then appropriate 3D printer and materials are carefully selected. The printer prints the scaffold as an output.
Types of 3D Printing
With the ever-increasing advancements of printing technology, there are multitudes of 3D printing techniques which are currently being used either in research or commercial applications. Some of the notable 3D printing technologies include stereolithography, inkjet printing, Fused Deposition Modeling (FDM), micro-extrusion, laser beam melting, Selective Laser Sintering (SLS), and Digital Laser Printing (DLP), electron beam melting, and polyjet printing [9]. Additionally, there are devices which utilise acoustic (ultrasound based), radio/microwave, Electro-hydrodynamic (EHD) and pneumatic based techniques for bioprinting of tissues and organs. Among these, inkjet printing, extrusion printing, laser assisted printing and stereolithography are some of the most widely adapted technologies in bioprinting [10]. Below sections briefly provide an overview of these approaches.
Extrusion Bioprinting
In simple words, extrusion bioprinting employs a process wherein a viscous bio-ink is passed through a small orifice to form a continuous cylindrical line and the same is printed in the substrate. Extrusion bioprinting can be of two types: pneumatic or mechanical extrusion printing, depending on the type of pneumatic or mechanical screw plungers employed, respectively; to dispense the bio-ink [Table/Fig-2a]. Extrusive bioprinting systems can utilise bio-ink with various viscosities, extrusion speed, procedure and deposit location can be flexibly adjusted [11]. Bioplotting or direct-writing system is a method which is based on extrusion bioprinting. Cells, cell encapsulated matrices and polymers can be used in bioplotting.
Schematic representations of some of the most commonly applied bioprinting techniques. (a) Extrusion based printing, (b) Inkjet printing, (c) Microwave-based printing, (d) Laser-assisted bioprinting.
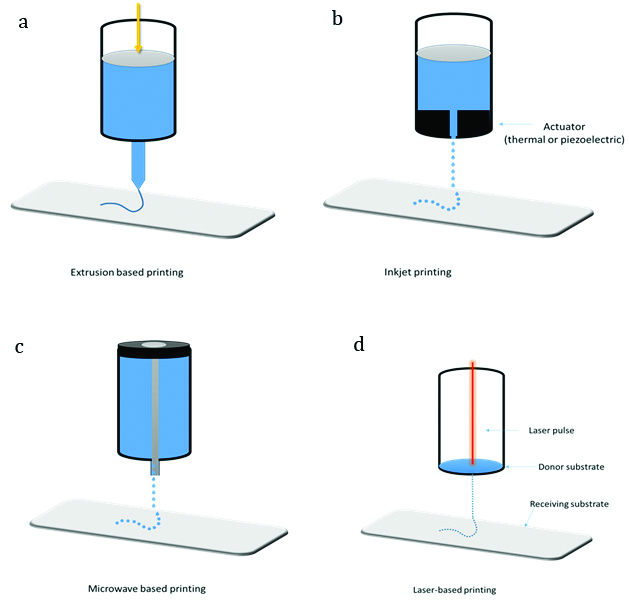
Ability to use high viscosity bio-inks and to utilise multiple printer heads with different bio-inks for complex printing requirements, and capable of handling relatively higher cell density are some key advantages of extrusion bioprinting. On the other hand, destruction of cell structure while passing through small printer head is one key disadvantage of extrusion bioprinting process [12].
Inkjet Printing
Inkjet printing was introduced as one of the first AM processes [13]. In simple words, the ink passes through a chamber which gets squeezed to expel the droplets from a pore [Table/Fig-2b]. Currently three main approaches namely thermal, piezoelectric and electrostatic are used for inkjet droplet squeezing. Of these, thermal and piezoelectric are the most widely utilised for structure fabrication [14]. Thermal inkjet printers eject the ink drops from the nozzles due to high pressure of air bubbles which are generated due to localised heating.
High speed, availability and low cost are three key advantages of inkjet printing. On the other hand, the major disadvantages include lack of precision in droplet placement and size, and dependency on low viscosity bioink [11]. Also, thermal inkjet printers are prone to clogging.
Stereolithography
Stereolithography is similar to the laser assisted bioprinting technique. Stereolithography printing uses Ultraviolet (UV) light to selectively solidify the photo-sensitive polymer, layer-by-layer and finally forms a complex structure. The organ structure is formatted in a corresponding stereolithography file which is converted from MRI and CT scan images. The structure is then sliced into thin layers and can be printed layer-by-layer. In stereolithography, the light solidifies the lower layer and corresponding top layers are overlapped to generate the 3D printed structure [15].
Enhanced fabrication accuracy and low printing time are two key advantages of stereolithography. On the other hand, lack of compatible materials, lengthy post-processing steps and use of high intensity UV light are some of the key disadvantages of Stereolithography [11].
Microvalve-based Droplet Ejection
Microvalve-based bioprinting system in general comprises a movable robotic platform and a multiple electromechanical microvalve print-heads. Each print-head is connected to a gas regulator that provides the pressure which opens the valve for a definite period of time. Movement of the plunger and solenoid coil regulate the opening time of the valve. A magnetic field is induced in the solenoid coil by applying a voltage, which causes opening of the nozzle. Eventually, the pneumatic pressure exceeds the resisting force in the nozzle orifice (fluid viscosity and surface tension), leading to deposition of the bio-ink [Table/Fig-2c]. Multiple factors like nozzle diameter, the viscosity and surface tension of the bio-ink, the pneumatic pressure and the valve opening time play an important role in material deposition process [16].
Laser-Assisted Printing
The origin of laser-assisted bioprinter is inspired from laser direct writing technique. Laser-assisted bioprinting of biomaterials is mainly treated by photo-polymerization method. Wide range of cells can be printed in this process and the cell viability is also well retained [12]. A focus tool redirects the laser beam from a pulsed laser source to a laser energy absorbing metallic ribbon film and a receiving substrate [Table/Fig-2d] [17]. During the printing, the laser focuses on the upper glass layer of the ribbon, coated with a nano-thickness gold and titanium film. The laser causes the film to evaporate, leading to formation of bubbles towards the bottom of the layer, which subsequently ejects the suspended bio-ink onto the receiving substrate as droplets.
Since laser assisted printing does not involves any nozzles, this technology has a potential to be applied to a wide variety of materials (e.g., hydrogels, ceramics, epoxy-based photoresist SU-8, cells and cell-encapsulate materials) [18]. Apart from high resolution printing, other advantages of laser-assisted bioprinting include high degree of precision, ability to use high viscosity printing and to print high cell density. On the other hand, the key disadvantages of this technique include high associated costs and time-consuming (lengthy) process [11].
Material/Cells in 3D Bioprinting
Since bioprinting involves biological system (e.g., cells, growth factors and scaffolds), the biomaterials should be non-cytotoxic, printable and also biodegradable in in vivo conditions to avoid secondary surgical removal of the implant, if needed. Additionally, the printed material should be firm enough to handle and also to assist the seeded cells [19]. It is desirable that the printed biomaterials should promote cell adhesion, maturation, migration, proliferation and differentiation [20].
The biopolymers can be briefly divided into three components-biomaterials, factors and cells/tissues [Table/Fig-3]. Biomaterial scaffolds are briefly natural and synthetic polymers. Natural polymers usually offer better biocompatibility and stable microenvironment for cells, as compared to synthetic polymers. On the other hand, natural polymers offer poor printability [21]. The common natural polymers used as biological materials include gelatin, collagen, alginate, chitosan, Hyaluronic Acid (HA) and agarose, whereas Polyethylene Glycol (PEG) and hydrogel are synthetic polymers [22] which have the relatively consistent chemical and mechanical properties to that of natural polymers. Hydrogels can absorb huge amount of biological fluids, and in swollen state they can be used to model living tissues as they appear to be soft and rubbery in nature. Due to their unique ability to allow diffusion of nutrients and oxygen, while offering adequate immune-isolation, hydrogels can play important role in tissue transplants [23] Additionally, synthetic polymers have better mechanical properties, and they usually are better than the natural ones [24] However, synthetic polymers also provide little or no niches for cell functions unlike natural polymers. However, high cell viability, better cell-cell interactions and tissue functionality are characteristics of natural polymers. [Table/Fig-4] provides a brief description of all three major polymers used.
Components of biopolymer.
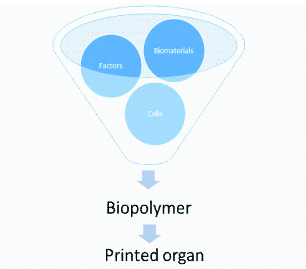
Overview of bioprinting scaffolds.
Description | Description | Printability | Advantage | Disadvantage | Examples |
---|
Natural | Sourced from biological metraials | Difficult | Highly biocompatible, good mechanical strength and stability, promotes adhesion, low cost | Difficult to modify as per specific needs, high chances of clogging | Agarose, gelatin, collagen, hyaluronic acid, matrigel |
Synthetic | Sourced from synthetic and natural sources | Easy | Can be easily modified to specific needs (functional groups), costly | Low biocompatibility | PEG based bioinks (PEG diacrylate) |
Hydrogel | Hydrophilic polymers as major component, Sourced from synthetic sources | Easy | Allow exchange of gases, nutrients, provide immunoisolation, viscosity can be adjusted | Poor mechanical properties, poor cell seeding, costly | Collagen or Keratin based hydrogels, poly (acrylic acid) sodium salt based hydrogels |
Cells are printed with appropriate biomaterials in a defined and organised manner in order make then highly mimetic to natural tissues. In order to facilitate the bio-printed cells to develop their in vivo functions, their immediate microenvironment should be able to mimic the physiological conditions in vivo. Multiple types of cells are required to play respective roles in the engineered tissues. When printing various types of cells for a complex tissue fabrication, these cells can be precisely seeded in their individual locations or in the same or different types of hydrogels simultaneously. Scientists have also tried to incorporate stem cells directly into scaffolds. Either the culture conditions, bioink additives, or stress induced during printing process can trigger stem cell differentiation [25] Moreover, since undifferentiated stem cells do not trigger immune response, they can also be used to avoid graft rejection after tissue transplantation.
Last but not the least, various factors (biomolecules) such as enzymes, growth factors, polynucleotides, polypeptides, as well as polysaccharides can be simultaneously printed with cells during tissue fabrication to manipulate cell proliferation, differentiation and migration in the printed subjects [26].
Applications of 3D Cell-Printing
Scientists have lot of expectations from 3D bioprinting technique as it has great potential in tissue engineering because of its flexibility and high resolution. Several tissue types like skin, bone, cartilage, liver, cardiovascular and neuronal tissues have already been generated using bioprinting techniques.
Blood Vessels
Tissue engineering has great potential to build a physiologically relevant and functionally active organ for those patients looking for a replacement organ for their lost or diseased body parts. Amongst all, the vascular system plays an important role for organ survival. Various approaches have been explored by researchers to create functional bio-printed vascular systems (e.g., in vivo and in vitro blood vessels) [27].
For example, in 2016, a group of scientists printed micro vascular structure using a modified commercially available inkjet bioprinter, human micro vascular endothelial cells and fibrin bio-ink [28]. Cell viability remains a major concern of vasculature bioprinting. Nevertheless, thermal inkjet bioprinters provide an optimal combination of cell viability while maintaining printing efficiency. Apart from cell viability, efficient crosslinking strategy is critical to fine tuning of tissues, physical properties and to shorten the printing process. Use of correct type of hydrogel could not only improve cell viability, but also aid in better cell spreading and proliferation.
Another research group able to develop thick prevascularized tissues comprising of layers of cells, and a decellularized Extracellular Matrix (dECM). The tissue also featured multi branched vasculature of different sizes [29]. The researchers also reported that cell viability and structural integrity was maintained even after 48 hours in in vitro conditions.
In a recent study, a bio-ink created by mixing photo-crosslinkable Gelatin Methacryloyl (GeIMA) with multiple cell types to mimic native vascular cell composition resulted in high cell viability (>85%) in vitro [30]. These bio-printed vascular constructs were found to be compatible and were integrated with host circulation. Further, in vivo subcutaneous transplantation revealed presence of red blood cells in the printed vascular constructs. This was an encouraging study and indicated the potential of bio-printed vasculature brought to the clinical use.
Apart from cell viability, the dimensions of bio-printed vasculature remain a key factor for its proper function. A group of scientists in 2009 came up with afully biological self-assembly approach, implemented through a rapid prototyping bioprinting method for scaffold-free small diameter (outer diameter: 0.9 to 2.5 mm) vascular reconstruction [31].
Similarly, another group reported rapid bioprinting method of aortic valve using sodium alginate supplemented Polyethylene Glycol Diacrylate (PEGDA) based hydrogels [32]. These scaffolds varied from 12 to 22 mm inner diameter, and exhibited cell adhesion as well as migration of porcine aortic valve interstitial cells. Some research labs are also working on a new type of filaments, known as sacrificial filaments (e.g., Pluronic F127), which can be used to form the fluidic channels. Such filaments not only aidthe process of vascular pattern creation but also could help to speed up the printing process [33-35].
Liver
Liver fibrosis is a major health issue. It develops over a period of time from a sequence of complex and cumulative interactions between hepatocytes and Non-parenchymal Cells (NPCs). Because of its complex nature, scientists found challenging to replicate it using in vitro and in vivo models.
One of the earlier bio-printed liver tissue models was made up of primary human hepatocytes, endothelial cells and hepatic stellate cells [36]. This model was targeted to mimic the basic fibrogenic features. This model was able to showcase that such cells can be incorporated in an automated and precise fashion. Same group in a recently published study modified the culture conditions leading to a gradual accumulation of collagen within the tissues over an extended exposure timeframe. Kupffer cells, when added to the model had a positive influence on TGF-β1 and methotrexate-induced fibrogenic response following cytokine and drug stimuli [37].
Another research group presented a proof-of-concept of bioprinting a liver organ by combining HepaRG cells with human stellate cells [38]. Using a stereolithographic printing approach, the bio-printed liver not only showed higher albumin and cytochrome P4503A4 expression over monolayer controls, but also the expression of tight junctions, as well as the indicators of overall metabolism (glucose, lactate, lactate dehydrogenase) were found to be stable under static cultivation conditions.
As per a recently published study, a group of scientists created a lung-mimicking hydrogel model of air sac where airways deliver oxygen to surrounding blood vessels [39]. Quick production time (few minutes) and efficient composition, which comprises intravascular 3D fluid mixersare the two major advantages of these transparent lung air sac hydrogel model. They also implanted structured hydrogel constructs containing liver cells into a rodent model of chronic liver injury and demonstrated the potential translational utility of such innovative biomaterials.
Cartilage
Cartilage tissue are different from other tissues as they donot contain nerves (aneural), or blood vessels (avascular). They receive the nutrients largely through synovial fluid [40]. Because of these typical differences, attempts of cartilage repair are not very successful [41]. Moreover, cartilage lesions fail to heal spontaneously, thus leading to chronic conditions which can reduce the quality of patient life. Just like surgical methods, tissue engineering approaches for cartilage repair also failed to construct new tissue that is similar to the native cartilage and possesses similar properties [42]. Inkjet bioprinting can be utilised to create tissues which closely mimic native tissues and thus can repair the cartilage without additional damage. A group of scientists printed human articulate chondrocytes embedded in a bio-ink to repair cartilage defects. These chondrocytes were evenly distributed in the hydrogel and exhibited better cell viabilities (up to 90%) [43]. Further, the printed cartilage implant was able to attach firmly with the surrounding tissues and increased production of extra cellular matrix was observed between the hydrogel scaffold and the host cartilage tissue. Another research groupwas able to print the ear cartilage, which was later successfully implanted subcutaneously in nude mice. Interestingly, the construct matured and formed a new cartilage formed throughout the entire construct after two months [44]. The above examples demonstrate the significance of direct cartilage repair using bioprinting technology.
The technique of cartilage regeneration utilises different scaffolds and printers. Poly-caprolactone and chondrocytes-encapsulated alginate hydrogel wasprinted with great biocompatibility using a multi-printhead system [45]. Additionally, amethod combining inkjet printing with electro spinning system was utilised for cartilage fabrication [46]. Electrospun PCL fibers were found to be integrated with inkjet printing of rabbit chondrocytes to create a five-layer tissue construct. The chondrocytes were found to be proliferated well within the printed constructs [41]. In addition, studies have also reported using alginate mixed with nano-cellulose to print auricular cartilage and meniscus constructs [47].
Muscle
Musculoskeletal injuries are quite common and can leave the patient weak and seriously affects patient’s ability to carry regular activities. Not only severe muscle injurylead to scar formation, but they might lead to chronic impairment if not healed properly [48]. The 3D bioprinting can have several advantages in muscle reconstruction.
In 2015, an integrated muscle-tendon unit was fabricated by printing four different components. It contained thermoplastic polyurethane and C2C12 myoblasts-laden hydrogel-based bio-ink for muscle development; and poly (ε-caprolactone) with NIH/3T3 cell-laden hydrogel for development of the tendon [49]. This method resulted in high cell viability with after seven days in vitro. Researchers have also printed myoblasts using a thermo inkjet printer and still retained high cell viability (>90%)[50]. Microcontact printing (also known as soft lithography method) has been used to print strips of fibronectin on biomaterial films for cell seeding [51].
Another group utilised layer-by-layer stereolithography technique to print muscle at higher resolutions [52]. Large actuation amplitudes of the specially designed printed device can mimic musculature function, but these constructs lack cell evidence.
Bone
Old age, infection, trauma and neoplasm might lead to bone injuries [53]. Common treatment approach to bone repair is allograft or xeno graft, but these processes have their own limitations like there are high chances of limited or no tissue supply, a majority of cases undergo secondary surgery, there is always a possibility of infection and immunological graft rejection [54]. Tissue engineering approaches like 3D bone printing, could provide promising solutions to overcome the limitations of traditional methods of bone repair.
Two important factors of any potential biomaterial to bone tissue printing include availability of proper biomaterials and appropriate cell types. Hydrogels have been found to show promising potential in bone regeneration. Bone tissue co-printingusing poly (ethylene glycol) dimethacrylate (PEGDMA) hydrogel and acrylated RGD and Matrix Metalloproteinase (MMP) peptides, resulted in a robust bone formation following peptide supplement [55]. Addition of bioactive glass nanoparticles in specific hydrogels could significantly improve the osteogenic differentiation of Mesenchymal Stem Cells (MSCs).
When hydrogel was replaced with a composite of PCL/PLGA/b-TCP membrane to induce bone regeneration, it significantly enhanced osteogenic differentiation using in vivo conditions [56].
Skin
Skin plays a pivotal role in providing protections from the external environment, as well as it helps to maintain homeostasis. Although there are many conventional skin-substitutes developed and being used in clinical practice, but these are not suitable for personalised skin treatment. These commercial skin-substitutes should be changed several times during the therapy which increases the overall complexity and cost of wound care.
Laser-assisted 3D bioprinting could be used to generate multicellular 3D constructs. These constructs contain fibroblasts, embedded in a collagen matrix with keratinocytes used as bio-ink [57]. The method of droplet-based bioprinting has been commonly used for skin tissue printing. Apart from collagen-based biomaterials, a recent modification of chitosan-based biomaterials was found suitable for functional 3D skin bioprinting. The chitosan-based method overcomes poor printability and speeds up the process as there is no prolonged collagen crosslinking time [58].
Vascularization is necessary to keep tissue alive, and thus, remains an important parameter for successful skin grafting. Cells can be printed on gels using inkjet devices and they exhibit high cell viability (>90%) [59]. In order to promote vascularization of skin implants, when researchers co-printed endothelial cells, keratinocytes and fibroblasts in the collagen matrix; it was observed that the combination favored cell survival and enhanced wound contraction [60]. However, the generation of new hair follicle or sweat gland growth in skin grafts remains critical challenge and needs more studies in future. As of now, no successful treatment strategies of generating human hair follicles has been reported. However, there is a hope that hair follicle growth needs hair neogenesis between the dermal papillae and epidermal cells [61]. It might be possible in near future to fabricate skin-mimicking constructs which have vasculature, nerve, hair follicles and sweat glands.
Neuronal Regeneration
Neuronal regeneration is another area of clinical practice where 3D bioprinting can display great potential. Recently, researchers used a piezoelectric inkjet printer to print porcine Schwann cells and NG108-15 cells for neural engineering [62]. The cell viability remained high despite high voltage in the printer and slight increase in cell viability was observed after seven days of printing.
Several in vivo studies have studied the impact of bioprinting in re-innervation or neuronal regeneration. For example, when photocurable polyethylene glycol resin was used with micro-stereolithography to print nerve guidance conduits [63], the conduits were able to support re-innervation across a 3 mm injury gap after three weeks. These results were comparable to that of an autograft control. In a recent paper, researchers reported a novel nerve guidance scaffold which jointly utilises fiber drawing with salt leaching to produce microchannels [64] The cross-section and porosity of these microchannels can be controlled, which is a major advantage in terms of large-scale process scalability. Another research group reported a customised way of nerve repair method which utilised microextrusion printing [65]. Alginate/CaCl2/PLGA/PCL/silicone/gelatin served as the printed materials. Gelatinmethacryloyl is a semi-synthetic hydrogel which contained either Nerve Growth Factor (NGF) or Glial Cell Line-derived Neurotrophic Factor (GDNF). Successful nerve regeneration was confirmed in printed scaffolds. Another research group bio-printed a biocompatible conduit using mouse marrow-derived stem cells, Schwann cells and agarose [66]. In this study, the printed graft was reinforced with a surrounding collagen tube. When implanted into a rat sciatic nerve injury model for 40 weeks, axon regrowth was observed in regions surrounding the agarose rods. The above examples largely provide pre-clinical evidence for the use of 3D bioprinting. A recent study formulated a bio-inspired filler free bioinks by selectively mixing natural polymers with synthetic polymers to obtain pre-defined rheological properties for the matrix. The free-standing cell laden tissue constructs were found to be biocompatible and exhibited minimal cytotoxic response during in vivo tests [67]. Such soft, free standing 3D neuronal tissues can be useful in the biofabrication of neural tissues for regenerative medicine.
Pancreas
Islet transplantation is considered as the preferred method for for Type 1 Diabates Mellitus (T1DM), although the chances of rejection from host-immune system are high [68]. If bio-printed pancreas is successful and commercialised, then pancreatic transplant would be a major treatment strategy for diabetes. The benefits of bio-printed pancreas include low rejection rate as the bio-printed pancreas would be built from patient’s own cells. Additionally, this will not only reduce patient waiting time, but will also reduce the economic burden of diabetes treatment.
Several researchers have tried to come up with practical strategies to place live islet cells in vascularised model of artificial pancreas [69]. Moreover, introducing regulatory T cells, endothelial progenitor cells and other similar cells to islet cells have demonstrated to improve the outcomes of transplantations [70].
Recently, a team of researchers from Poland was able to create the first 3D printed prototype of a vascularised bionic pancreas. This pancreas is made up of pancreatic islets producing glucagon and insulin and has been successfully evaluated in flow-connected bioreactor. Stem cells were extracted from a patient and were converted to alpha and beta cells capable of producing glucagon and insulin. These cells were then mixed with a specially formulated bio-ink and a pancreatic scaffold was produced. The same scaffold also had elements which were bio-printed to replicate pancreatic vascular system.
Researchers used MRI and CT tests to confirm that the internal vascular structure of the bio-printed organ perfectly matched with the prototype [71]. The next step would be to conduct animal studies to gain valuable insights about how bio-printed pancreas behaves inside a living organism.
In a recent study, researchers were able to fabricate 3D structures containing macroporous hydrogel constructs with embedded islet cells [72]. They achieved this by combining islet encapsulation with 3D extrusion bioprinting. The construct retained its viability, morphology, and functionality throughout the study duration. The researchers also reported continuous insulin production by embedded islets. Therefore, to an extent, it is possible to create 3D bioprinted pancreas which have potential to play important role in clinical management of T1DM.
Recent Advancements
An interesting study reported vascularisation and ectopic bone formation in the porous scaffolds seeded with co-cultured Human Umbilical Vein Endothelial Cells (HUVECs). The study investigated the effects of Human-Induced Pluripotent Stem Cell-Derived (hiPSC-MSCs) co-cultured with HUVECs for prevascularisation of Calcium Phosphate Cement (CPC) scaffold on bone regeneration in vivo for the first time. The study concluded that HUVECs, when co-cultured with hiPSC-MSCs substantially promoted bone regeneration. The novel construct of HUVECs co-cultured with hiPSC-MSCs delivered via CPC scaffolds seemed promising to enhance bone and vascular regeneration in orthopedic settings [73].
In an interesting research, scientists createdbio prosthetic ovary using 3D printed microporous scaffolds which restored ovarian function in sterilised mice [74]. The scaffolds were initially follicle-seeded, eventually became highly vascularized and ovarian function was fully restored when they were implanted in surgically sterilised mice. Interestingly, pups were born through natural mating and thrived through maternal lactation.
The meniscus is a complex, c-shaped cartilage in the knee and acts as shock absorber at the knee joint. Degenerative meniscal tear often leads to arthritis. In this interesting study, scientists reported the regeneration of an inhomogeneous, functional knee meniscus in a large animal model (sheep) with 3-month follow-up [75]. They replaced sheep meniscus with anatomically correct, 3D–printed scaffolds. The study reported that inhomogeneous mechanical properties were restored in the regenerated sheep meniscus. This study further highlighted the importance of survival and differentiation of endogenous cells in a tissue, as tissue defect might affect the regeneration of complex tissues.
Many research groups are working towards development and optimisation of a 3D on-demand cell and protein printing platform, considering human skin as a prototype A group of researchers followed a new 3D cell-printing strategy and were able to create a skin model with a functional trans well system [76]. This model had a stabilised fibroblast-stretched dermis and it was able to retain stratified epidermis layers even after 14 days. Moreover, this method was also found to be both, cost effective as well as utilised less resources than a conventional culture.
A minimal effect on cell viability and function has been reported when cells and proteins were printed in nano-or microliter droplets on planar surfaces [77]. Researchers used keratinocytes and fibroblasts as constituent cells to represent the epidermis and dermis layer, and collagen to represent the dermal matrix of the skin. They found only few dissimilarities between 3D printed skin was and biological representative of in vivo human skin tissue.
Another research group have reportedly generated a 3D printed bionic ear which is made up of a cell-seeded hydrogel matrix and is in the shape of a human ear. Apart from listening to stereo sounds (separate sound reception through left and right ear), the printed ear can also hear radio frequency reception, which is beyond the capability of normal human ear [78]. This bionic ear also contains a conducting polymer of infused silver nanoparticles. In vitro cartilage tissue culturing of around an inductive coil antenna consequently enabled detection of inductive signals from electrodes.
Development in India
With its immense potential in design and development, 3D printing has emerged in recent years as a technology that can change the world of clinical healing. Though the 3D bioprinting technology itself is in its nascent stage, there are some Indian companies which are offering 3D printing services to the Indian Medical Community.
Bangalore based Pandorum Technologies is a renowned Indian bio-tech start-up company which has successfully developed artificial 3D bio-printed liver tissues. Since these tissues somewhat mimic human liver functions, they can not only offer a low-cost model of medical research for medicines and vaccines, but also can be used for full-scale, 3D printed organs. Pandorum had also received success in developing human cornea to enable scar-less healing of corneal wounds and help restore vision through bio-regeneration [79].
Similarly, Gurugram based Nova beans provides facial reconstruction surgery for cancer patients [80]. Apart from these, they also provide digital dentistry solutions, skull reconstruction surgery, spinal cord injury models and brain aneurysm surgery models. They aimed to bring on-demand affordable 3D printed solutions to cater Indian healthcare industry.
Likewise, Mumbai based Anatomis 3d is working on 3D printed parts of the heart [81] and had published papers on 3D printed cardiac proto types and models [82-84].
Another Bangalore based company named Next Big Innovation Labs (NBIL) Pvt., Ltd., had developed India’s first customisable 3D Bioprinter named ‘Trivima’, and they aimed to offer this as a service for researchers [85]. Apart from this, they are working on the final stages of 3D Bio-printed human skin ‘Innoskin’ which is the first of its kind in India and uses the patented 3D Bioprinting technology developed by the NBIL team. Innoskin has two testing variants – Human Epidermis (HE) and FT (dermal and epidermal model, together). The company is planning to offer its products to treat burns, wounds and other skin related patients.
Last but not the least, a team of scientists from Department of Textile technology, IIT-Delhi has developed a 3D bio-printed cartilage that is remarkably similar to the natural ones seen in human knees [86]. As per the research group, this was the first 3D bio-printed tissue which has been developed in a lab in India.
Limitation
Despite the success stories and reported outstanding research efforts, there are several challenges to be addressed before having a fully built 3D bio-printed organ. The first and foremost problem is need to increase the speed of bioprinting. The current printing process is slow to reach a scalable and commercially acceptable level, 3D bioprinting needs to identify materials which can help faster printing. The next challenge is to increase the resolution of bio-printed constructs, which is followed by compatibility issues with a larger spectrum of biocompatible materials. Higher resolution will not only enable better material interaction, but it will also provide better control in 3D microenvironment. On the other hand, identification of synthetic biomaterials can help to address these two issues. A blend of biopolymers which are biocompatible and provide strength to the construct is needed.
Additionally, vasculature of the construct should be sufficient enough to allow oxygen and nutrient diffusion, as well as elimination of cellular and metabolic wastes. This can be ensured by incorporating vasculature in the early stages of the construct, or by adding angiogenic factors to the construct so that the vasculature can be formed during the later stages. Apart from these, safety, legal and ethical problems might arise due course of this technology, hence, these aspects need to be explored before it undergoes large-scale production.
Future Perspectives
The 3D printing is new, yet challenging tool which has lots of promising potential to offer to the medical field. This has to be considered as a revolution in the medical field, as the 3D printed organs have potential to ease the burden and shortage of organ transplants. It will also help to reduce the healthcare and associated costs as the organ will be available on time reducing the waiting period. Reduced shortage of organs can help to decrease the death rate for some chronic diseases. Additionally, the rate of a successful transplant will increase as the rate of rejection will go down. This will also reduce associated cost as the patient will be hospitalised for fewer days with no need of anti-rejection drugs. Most importantly, 3D printed organs will bring an era of personalised medicine where transplanted tissues will be engineered as per the patient’s needs. Overall, the 3D organ printing will revolutionise regenerative medicine.
Although tissue engineering looks promising, it is still in a nascent state and there is a long way to go to make it a complete dependable technology on its own. Despite of limitations like high cost of research and production, and the lack of proper infrastructure, the increasing demand of organ transplants and associated higher graft rejection rates, technological advancements are some of the factors boosting the 3D cell culture market globally. Recent technological advancements towards development of automated large-scale cell cultures [87], innovations inbioprocess engineering, implementation and incorporation of interdisciplinary techniques (e.g., incorporation of genetic tools to direct cell fate), are encouraging [88]. These advancements indicate that in near future, researchers will be able to create affordable patient-specific high precision 3D printed structures, which is not possible today.
It also makes sense to obtain diseased tissue or cells from a patient and subject these samples to gene editing. The gene edited cells could be possibly used to achieve a prespecified endpoint(s) [89]. Similarly, an array of various sets of gene-edited tissues can be printed together to achieve a broader endpoint [89]. Such unconventional bioprinting approaches when used with interdisciplinary tools (e.g., gene editing) can bring unprecedented advancements in the field of regenerative medicine and tissue engineering.
Conclusion
Like any new technology, the 3D printing has introduced unlimited possibilities for the medical field, but this will also include a clause of diversity as each patient’s need will be clinically different. This will surely bring the era of personalised medicine. However, this should be monitored closely by some regulatory body to avoid misuse of the technology.
[1]. Xiong R, Zhang Z, Huang Y, Identification of optimal printing conditions for laser printing of alginate tubular constructs J Manuf Process 2015 20(3):450-55.10.1016/j.jmapro.2015.06.023 [Google Scholar] [CrossRef]
[2]. Miranda B, Matesanz R, International issues in transplantation: setting the scene and flagging the most urgent and controversial issues Ann N Y Acad Sci 1998 862:129-43.10.1111/j.1749-6632.1998.tb09126.x9928215 [Google Scholar] [CrossRef] [PubMed]
[3]. Li P, Zhang W, Smith LJ, Ayares D, Cooper DK, Ekser B, The potential role of 3D-bioprinting in xenotransplantation Curr Opinion Org Trransplant 2019 doi: 10.1097/MOT.0000000000000684. [Epub ahead of print]10.1097/MOT.000000000000068431385888 [Google Scholar] [CrossRef] [PubMed]
[4]. Organ donation statistics. Available from https://www.organdonor.gov/statistics-stories/statistics.html. Accessed on 26th July 2019 [Google Scholar]
[5]. Hull CW, inventor; UVP Inc, assignee. Apparatus for production of three-dimensional objects by stereolithography. United States patent US 4,575,330. 1986 Mar 11. Available from: https://patents.google.com/patent/US4575330A/en. Accessed on 26th July 2019 [Google Scholar]
[6]. Wu Z, Su X, Xu Y, Kong B, Sun W, Mi S, Bioprinting three-dimensional cell-laden tissue constructs with controllable degradation Sci Rep 2016 6:2447410.1038/srep2447427091175 [Google Scholar] [CrossRef] [PubMed]
[7]. Gu BK, Choi DJ, Park SJ, Kim YJ, Kim CH, 3D bioprinting technologies for tissue engineering applications Adv Exp Med Biol 2018 1078:15-28.10.1007/978-981-13-0950-2_230357616 [Google Scholar] [CrossRef] [PubMed]
[8]. Eswaramoorthy SD, Ramakrishna S, Rath SN, Recent advances in three-dimensional bioprinting of stem cells J Tissue Eng Regen Med 2019 13(6):908-24.10.1002/term.283930866145 [Google Scholar] [CrossRef] [PubMed]
[9]. Han T, Kundu S, Nag A, Xu Y, 3D printed sensors for biomedical applications: A review Sensors 2019 19(7):170610.3390/s1907170630974757 [Google Scholar] [CrossRef] [PubMed]
[10]. Huang Y, Zhang XF, Gao G, Yonezawa T, Cui X, 3D bioprinting and the current applications in tissue engineering Biotechnology journal 2017 12(8):160073410.1002/biot.20160073428675678 [Google Scholar] [CrossRef] [PubMed]
[11]. Shafiee A, Atala A, Printing technologies for medical applications Trends in molecular medicine 2016 22(3):254-65.10.1016/j.molmed.2016.01.00326856235 [Google Scholar] [CrossRef] [PubMed]
[12]. Bishop ES, Mostafa S, Pakvasa M, Luu HH, Lee MJ, Wolf JM, 3-D bioprinting technologies in tissue engineering and regenerative medicine: Current and future trends Genes Dis 2017 4(4):185-95.10.1016/j.gendis.2017.10.00229911158 [Google Scholar] [CrossRef] [PubMed]
[13]. Derby B, Additive manufacture of ceramics components by inkjet printing Engineering 2015 1(1):113-23.10.15302/J-ENG-2015014 [Google Scholar] [CrossRef]
[14]. Ihalainen P, Määttänen A, Sandler N, Printing technologies for biomolecule and cell-based applications Int J Pharm 2015 494(2):585-92.10.1016/j.ijpharm.2015.02.03325683144 [Google Scholar] [CrossRef] [PubMed]
[15]. Brunello G, Sivolella S, Meneghello R, Ferroni L, Gardin C, Piattelli A, Powder-based 3D printing for bone tissue engineering Biotechnol Adv 2016 34(5):740-53.10.1016/j.biotechadv.2016.03.00927086202 [Google Scholar] [CrossRef] [PubMed]
[16]. Lee W, Debasitis JC, Lee VK, Lee JH, Fischer K, Edminster K, Multi-layered culture of human skin fibroblasts and keratinocytes through three-dimensional freeform fabrication Biomaterials 2009 30(8):1587-95.10.1016/j.biomaterials.2008.12.00919108884 [Google Scholar] [CrossRef] [PubMed]
[17]. Koch L, Gruene M, Unger C, Chichkov B, Laser assisted cell printing Curr Pharm Biotechnol 2013 14(1):91-97.10.2174/13892011380480536823570054 [Google Scholar] [CrossRef] [PubMed]
[18]. Jana S, Lerman A, Bioprinting a cardiac valve Biotechnol Adv 2015 33(8):1503-21.10.1016/j.biotechadv.2015.07.00626254880 [Google Scholar] [CrossRef] [PubMed]
[19]. Jammalamadaka U, Tappa K, Recent advances in biomaterials for 3D printing and tissue engineering J Funct Biomate 2018 9(1):2210.3390/jfb901002229494503 [Google Scholar] [CrossRef] [PubMed]
[20]. Arealis G, Nikolaou VS, Bone printing: New frontiers in the treatment of bone defects Injury 2015 46(Suppl 8):S20-22.10.1016/S0020-1383(15)30050-4 [Google Scholar] [CrossRef]
[21]. An J, Teoh JE, Suntornnond R, Chua CK, Design and 3D printing of scaffolds and tissues Engineering 2015 1(2):261-68.10.15302/J-ENG-2015061 [Google Scholar] [CrossRef]
[22]. Tappa K, Jammalamadaka U, Novel biomaterials used in medical 3D printing techniques J Funct Biomater 2018 9(1):1710.3390/jfb901001729414913 [Google Scholar] [CrossRef] [PubMed]
[23]. El-Sherbiny IM, Yacoub MH, Hydrogel scaffolds for tissue engineering: Progress and challenges Glob Cardiol Sci Pract 2013 2013(3):316-42.10.5339/gcsp.2013.3824689032 [Google Scholar] [CrossRef] [PubMed]
[24]. Gopinathan J, Noh I, Recent trends in bioinks for 3D printing Biomater Res 2018 22:1110.1186/s40824-018-0122-129636985 [Google Scholar] [CrossRef] [PubMed]
[25]. Irvine S, Venkatraman S, Bioprinting and differentiation of stem cells Molecules 2016 21(9):118810.3390/molecules2109118827617991 [Google Scholar] [CrossRef] [PubMed]
[26]. Cui X, Lu YW, Lee V, Kim D, Dorsey T, Wang Q, Venous endothelial marker COUP-TFII regulates the distinct pathologic potentials of adult arteries and veins Sci Rep 2015 5:1619310.1038/srep1619326537113 [Google Scholar] [CrossRef] [PubMed]
[27]. Jafarkhani M, Salehi Z, Aidun A, Shokrgozar MA, Bioprinting in vascularization strategies Iran Biomed J 2019 23(1):910.29252/ibj.23.1.930458600 [Google Scholar] [CrossRef] [PubMed]
[28]. Jia W, Gungor-Ozkerim PS, Zhang YS, Yue K, Zhu K, Liu W, Direct 3D bioprinting of perfusable vascular constructs using a blend bioink Biomaterials 2016 06:58-68.10.1016/j.biomaterials.2016.07.03827552316 [Google Scholar] [CrossRef] [PubMed]
[29]. Xu Y, Hu Y, Liu C, Yao H, Liu B, Mi S, A novel strategy for creating tissue-engineered biomimetic blood vessels using 3D bioprinting technology Materials 2018 11(9):158110.3390/ma1109158130200455 [Google Scholar] [CrossRef] [PubMed]
[30]. Zhu W, Qu X, Zhu J, Ma X, Patel S, Liu J, Direct 3D bioprinting of prevascularized tissue constructs with complex microarchitecture Biomaterials 2017 124:106-15.10.1016/j.biomaterials.2017.01.04228192772 [Google Scholar] [CrossRef] [PubMed]
[31]. Norotte C, Marga FS, Niklason LE, Forgacs G, Scaffold-free vascular tissue engineering using bioprinting Biomaterials 2009 30(30):5910-17.10.1016/j.biomaterials.2009.06.03419664819 [Google Scholar] [CrossRef] [PubMed]
[32]. Hockaday LA, Kang KH, Colangelo NW, Cheung PY, Duan B, Malone E, Rapid 3D printing of anatomically accurate and mechanically heterogeneous aortic valve hydrogel scaffolds Biofabrication 2012 4(3):03500510.1088/1758-5082/4/3/03500522914604 [Google Scholar] [CrossRef] [PubMed]
[33]. Wu W, DeConinck A, Lewis JA, Omnidirectional printing of 3D microvascular networks Adv Mater 2011 23(24):H178-83.10.1002/adma.20100462521438034 [Google Scholar] [CrossRef] [PubMed]
[34]. Kolesky DB, Truby RL, Gladman AS, Busbee TA, Homan KA, Lewis JA, 3D bioprinting of vascularized, heterogeneous cell-laden tissue constructs Adv Mater 2014 26(19):3124-30.10.1002/adma.20130550624550124 [Google Scholar] [CrossRef] [PubMed]
[35]. Lee W, Lee V, Polio S, Keegan P, Lee JH, Fischer K, On demand three dimensional freeform fabrication of multi layered hydrogel scaffold with fluidic channels Biotechnol Bioeng 2010 105(6):1178-86.10.1002/bit.2261319953677 [Google Scholar] [CrossRef] [PubMed]
[36]. Norona LM, Nguyen DG, Gerber DA, Presnell SC, LeCluyse EL, Editor’s highlight: modeling compound-induced fibrogenesis in vitro using three-dimensional bioprinted human liver tissues Toxicol Sci 2016 154(2):354-67.10.1093/toxsci/kfw16927605418 [Google Scholar] [CrossRef] [PubMed]
[37]. Norona LM, Nguyen DG, Gerber DA, Presnell SC, Mosedale M, Watkins PB, Bioprinted liver provides early insight into the role of Kupffer cells in TGF-β1 and methotrexate-induced fibrogenesis PloS one 2019 14(1):e020895810.1371/journal.pone.020895830601836 [Google Scholar] [CrossRef] [PubMed]
[38]. Grix T, Ruppelt A, Thomas A, Amler AK, Noichl B, Lauster R, Bioprinting perfusion-enabled liver equivalents for advanced organ-on-a-chip applications Genes 2018 9(4):17610.3390/genes9040176 [Google Scholar] [CrossRef]
[39]. Grigoryan B, Paulsen SJ, Corbett DC, Sazer DW, Fortin CL, Zaita AJ, Multivascular networks and functional intravascular topologies within biocompatible hydrogels Sci 2019 364(6439):458-64.10.1126/science.aav975031048486 [Google Scholar] [CrossRef] [PubMed]
[40]. Minas T, A primer in cartilage repair J Bone Joint Surg Br 2012 94(11 Supple A):141-46.10.1302/0301-620X.94B11.3067923118403 [Google Scholar] [CrossRef] [PubMed]
[41]. McCormick F, Harris JD, Abrams GD, Frank R, Gupta A, Hussey K, Trends in the surgical treatment of articular cartilage lesions in the United States: An analysis of a large private-payer database over a period of 8 years Arthroscopy 2014 30(2):222-26.10.1016/j.arthro.2013.11.00124485115 [Google Scholar] [CrossRef] [PubMed]
[42]. Hunziker EB, Articular cartilage repair: Basic science and clinical progress. A review of the current status and prospects Osteoarthritis Cartilage 2002 10(6):432-63.10.1053/joca.2002.080112056848 [Google Scholar] [CrossRef] [PubMed]
[43]. Cui X, Breitenkamp K, Finn MG, Lotz M, D’Lima DD, Direct human cartilage repair using three-dimensional bioprinting technology Tissue Eng Part A 2012 18(11-12):1304-12.10.1089/ten.tea.2011.054322394017 [Google Scholar] [CrossRef] [PubMed]
[44]. Kang HW, Lee SJ, Ko IK, Kengla C, Yoo JJ, Atala A, A 3D bioprinting system to produce human-scale tissue constructs with structural integrity Nat Biotechnol 2016 34(3):31210.1038/nbt.341326878319 [Google Scholar] [CrossRef] [PubMed]
[45]. Kundu J, Shim JH, Jang J, Kim SW, Cho DW, An additive manufacturing based PCL–alginate–chondrocyte bioprinted scaffold for cartilage tissue engineering J Tissue Eng Regen Med 2015 9(11):286-97.10.1002/term.168223349081 [Google Scholar] [CrossRef] [PubMed]
[46]. Xu T, Binder KW, Albanna MZ, Dice D, Zhao W, Yoo JJ, Hybrid printing of mechanically and biologically improved constructs for cartilage tissue engineering applications Biofabrication 2012 5(1):01500110.1088/1758-5082/5/1/01500123172542 [Google Scholar] [CrossRef] [PubMed]
[47]. Markstedt K, Mantas A, Tournier I, Martínez Ávila H, Hägg D, Gatenholm P, 3D bioprinting human chondrocytes with nanocellulose-alginate bioink for cartilage tissue engineering applications Biomacromolecules 2015 16(5):489-96.10.1021/acs.biomac.5b0018825806996 [Google Scholar] [CrossRef] [PubMed]
[48]. Evans CH, Huard J, Gene therapy approaches to regenerating the musculoskeletal system Nat Rev Rheumatol 2015 11(4):23410.1038/nrrheum.2015.2825776949 [Google Scholar] [CrossRef] [PubMed]
[49]. Merceron TK, Burt M, Seol YJ, Kang HW, Lee SJ, Yoo JJ, A 3D bioprinted complex structure for engineering the muscle-tendon unit Biofabrication 2015 7(3):03500310.1088/1758-5090/7/3/03500326081669 [Google Scholar] [CrossRef] [PubMed]
[50]. Cui X, Gao G, Qiu Y, Accelerated myotube formation using bioprinting technology for biosensor applications Biotechnol Lett 2013 35(3):315-21.10.1007/s10529-012-1087-023160742 [Google Scholar] [CrossRef] [PubMed]
[51]. Altomare L, Riehle M, Gadegaard N, Tanzi M, Farè S, Microcontact printing of fibronectin on a biodegradable polymeric surface for skeletal muscle cell orientation Int J Artif Organs 2010 33(8):535-43.10.1177/03913988100330080420872348 [Google Scholar] [CrossRef] [PubMed]
[52]. Peele BN, Wallin TJ, Zhao H, Shepherd RF, 3D printing antagonistic systems of artificial muscle using projection stereolithography Bioinspir Biomim 2015 10(5):05500310.1088/1748-3190/10/5/05500326353071 [Google Scholar] [CrossRef] [PubMed]
[53]. Reichert JC, Saifzadeh S, Wullschleger ME, Epari DR, Schütz MA, Duda GN, The challenge of establishing preclinical models for segmental bone defect research Biomaterials 2009 30(12):2149-63.10.1016/j.biomaterials.2008.12.05019211141 [Google Scholar] [CrossRef] [PubMed]
[54]. Mouriño V, Boccaccini AR, Bone tissue engineering therapeutics: controlled drug delivery in three-dimensional scaffolds J R Soc Interface 2009 7(43):209-27.10.1098/rsif.2009.037919864265 [Google Scholar] [CrossRef] [PubMed]
[55]. Gao G, Schilling AF, Yonezawa T, Wang J, Dai G, Cui X, Bioactive nanoparticles stimulate bone tissue formation in bioprinted three-dimensional scaffold and human mesenchymal stem cells Biotechnol J 2014 9(10):1304-11.10.1002/biot.20140030525130390 [Google Scholar] [CrossRef] [PubMed]
[56]. Pati F, Song TH, Rijal G, Jang J, Kim SW, Cho DW, Ornamenting 3D printed scaffolds with cell-laid extracellular matrix for bone tissue regeneration Biomaterials 2015 37:230-41.10.1016/j.biomaterials.2014.10.01225453953 [Google Scholar] [CrossRef] [PubMed]
[57]. Koch L, Deiwick A, Schlie S, Michael S, Gruene M, Coger V, Skin tissue generation by laser cell printing Biotechnol Bioeng 2012 109(7):1855-63.10.1002/bit.2445522328297 [Google Scholar] [CrossRef] [PubMed]
[58]. Ng WL, Yeong WY, Naing MW, Polyelectrolyte gelatin-chitosan hydrogel optimized for 3D bioprinting in skin tissue engineering Int J Bioprint 2016 2(1):53-62.10.18063/IJB.2016.01.009 [Google Scholar] [CrossRef]
[59]. Cui X, Boland T, DD’Lima D, K Lotz M, Thermal inkjet printing in tissue engineering and regenerative medicine Recent patents on drug delivery & formulation 2012 6(2):149-55.10.2174/18722111280067294922436025 [Google Scholar] [CrossRef] [PubMed]
[60]. Yanez M, Rincon J, Dones A, De Maria C, Gonzales R, Boland T, In vivo assessment of printed microvasculature in a bilayer skin graft to treat full-thickness wounds Tissue Eng Part A 2014 21(1-2):224-33.10.1089/ten.tea.2013.056125051339 [Google Scholar] [CrossRef] [PubMed]
[61]. Ng WL, Wang S, Yeong WY, Naing MW, Skin bioprinting: impending reality or fantasy? Trends in Biotechnol 2016 34(9):689-99.10.1016/j.tibtech.2016.04.00627167724 [Google Scholar] [CrossRef] [PubMed]
[62]. Tse C, Whiteley R, Yu T, Stringer J, MacNeil S, Haycock JW, Inkjet printing Schwann cells and neuronal analogue NG108-15 cells Biofabrication 2016 8(1):01501710.1088/1758-5090/8/1/01501726930268 [Google Scholar] [CrossRef] [PubMed]
[63]. Pateman CJ, Harding AJ, Glen A, Taylor CS, Christmas CR, Robinson PP, Nerve guides manufactured from photocurable polymers to aid peripheral nerve repair Biomaterials 2015 49:77-89.10.1016/j.biomaterials.2015.01.05525725557 [Google Scholar] [CrossRef] [PubMed]
[64]. Shahriari D, Loke G, Tafel I, Park S, Chiang PH, Fink Y, Anikeeva P, Scalable Fabrication of Porous Microchannel Nerve Guidance Scaffolds with Complex Geometries Adv Mater 2019 31(30):e190202110.1002/adma.20190202131168865 [Google Scholar] [CrossRef] [PubMed]
[65]. Johnson BN, Lancaster KZ, Zhen G, He J, Gupta MK, Kong YL, 3D printed anatomical nerve regeneration pathways Adv Funct Mater 2015 25(39):6205-17.10.1002/adfm.20150176026924958 [Google Scholar] [CrossRef] [PubMed]
[66]. Owens CM, Marga F, Forgacs G, Heesch CM, Biofabrication and testing of a fully cellular nerve graft Biofabrication 2013 5(4):04500710.1088/1758-5082/5/4/04500724192236 [Google Scholar] [CrossRef] [PubMed]
[67]. Haring AP, Thompson EG, Tong Y, Laheri S, Cesewski E, Sontheimer H, Process-and bio-inspired hydrogels for 3D bioprinting of soft free-standing neural and glial tissues Biofabrication 2019 11(2):02500910.1088/1758-5090/ab02c930695770 [Google Scholar] [CrossRef] [PubMed]
[68]. Rother KI, Harlan DM, Challenges facing islet transplantation for the treatment of type 1 diabetes mellitus The Journal of Clinical Investigation 2004 114(7):877-83.10.1172/JCI200423235 [Google Scholar] [CrossRef]
[69]. Lee SJ, Lee JB, Park YW, Lee DY, 3D bioprinting for artificial pancreas organ Adv Exp Med Biol 2018 1064:355-74.10.1007/978-981-13-0445-3_2130471043 [Google Scholar] [CrossRef] [PubMed]
[70]. Kim J, Kang K, Drogemuller CJ, Wallace GG, Coates PT, Bioprinting an artificial pancreas for Type 1 diabetes Curr Diab Rep 2019 19(8):5310.1007/s11892-019-1166-x31273530 [Google Scholar] [CrossRef] [PubMed]
[71]. Polish Fundacja moves ahead with 3D bioprinted bionic pancreas. Available from: project https://www.3dprintingmedia.network/polish-fundacja-3d-bioprinted-bionic-pancreas/. Accessed on 26th July 2019 [Google Scholar]
[72]. Duin S, Schütz K, Ahlfeld T, Lehmann S, Lode A, Ludwig B, Gelinsky M, 3D bioprinting of functional islets of langerhans in an alginate/Methylcellulose hydrogel blend Adv Healthc Mater 2019 8(7):180163110.1002/adhm.20180163130835971 [Google Scholar] [CrossRef] [PubMed]
[73]. Liu X, Chen W, Zhang C, Thein-Han W, Hu K, Reynolds MA, Co-seeding human endothelial cells with human-induced pluripotent stem cell-derived mesenchymal stem cells on calcium phosphate scaffold enhances osteogenesis and vascularization in rats Tissue Eng Part A 2017 23(11-12):546-55.10.1089/ten.tea.2016.048528287922 [Google Scholar] [CrossRef] [PubMed]
[74]. Laronda MM, Rutz AL, Xiao S, Whelan KA, Duncan FE, Roth EW, A bioprosthetic ovary created using 3D printed microporous scaffolds restores ovarian function in sterilized mice Nature communications 2017 8:1526110.1038/ncomms1526128509899 [Google Scholar] [CrossRef] [PubMed]
[75]. Lee CH, Rodeo SA, Fortier LA, Lu C, Erisken C, Mao JJ, Protein-releasing polymeric scaffolds induce fibrochondrocytic differentiation of endogenous cells for knee meniscus regeneration in sheep Sci Transl Med 2014 6(266):266ra17110.1126/scitranslmed.300969625504882 [Google Scholar] [CrossRef] [PubMed]
[76]. Kim BS, Lee JS, Gao G, Cho DW, Direct 3D cell-printing of human skin with functional transwell system Biofabrication 2017 9(2):02503410.1088/1758-5090/aa71c828586316 [Google Scholar] [CrossRef] [PubMed]
[77]. Lee V, Singh G, Trasatti JP, Bjornsson C, Xu X, Tran TN, Design and fabrication of human skin by three-dimensional bioprinting Tissue Eng Part C: Methods 2013 20(6):473-84.10.1089/ten.tec.2013.033524188635 [Google Scholar] [CrossRef] [PubMed]
[78]. Mannoor MS, Jiang Z, James T, Kong YL, Malatesta KA, Soboyejo WO, 3D printed bionic ears Nano Lett 2013 13(6):2634-39.10.1021/nl400774423635097 [Google Scholar] [CrossRef] [PubMed]
[79]. Building blocks of life: Tissue Engineering & Regenerative Medicine. Available from: http://www.pandorumtechnologies.com. Accessed on 26th July 2019 [Google Scholar]
[80]. Medical 3D Printing: Additive Manufacturing. Available from: http://www.novabeans.com/medical/. Accessed on 26th July 2019 [Google Scholar]
[81]. Medical designs and 3D painting solutions Bureau. Available from: https://anatomiz3d.com. Accessed on 26th July 2019 [Google Scholar]
[82]. Garekar S, Bharati A, Chokhandre M, Mali S, Trivedi B, Changela VP, Clinical application and multidisciplinary assessment of three dimensional printing in double outlet right ventricle with remote ventricular septal defect World J Pediatr Congenit Heart Surg 2016 7(3):344-50.10.1177/215013511664560427142402 [Google Scholar] [CrossRef] [PubMed]
[83]. Bharati A, Garekar S, Agarwal V, Merchant SA, Solanki N, MRA-based 3D-printed heart model-an effective tool in the pre-surgical planning of DORV BJR Case Rep 2016 2(3):2015043610.1259/bjrcr.2015043630459986 [Google Scholar] [CrossRef] [PubMed]
[84]. Kappanayil M, Koneti NR, Kannan RR, Kottayil BP, Kumar K, Three-dimensional-printed cardiac prototypes aid surgical decision-making and preoperative planning in selected cases of complex congenital heart diseases: early experience and proof of concept in a rei-limited environment Ann Pediatr Cardiol 2017 10(2):117-25.10.4103/apc.APC_149_1628566818 [Google Scholar] [CrossRef] [PubMed]
[85]. Trivima, India’s first customisable 3D Bioprinter. Next Big Innovation Labs (NBIL) Pvt. Ltd. Available from: https://nextbiglab.com/. Accessed on 26th July 2019 [Google Scholar]
[86]. Chawla S, Kumar A, Admane P, Bandyopadhyay A, Ghosh S, Elucidating role of silk-gelatin bioink to recapitulate articular cartilage differentiation in 3D bioprinted constructs Bioprinting 2017 7:01-03.10.1016/j.bprint.2017.05.001 [Google Scholar] [CrossRef]
[87]. 3D Cell Culture Market Report, Size, Share, Analysis 2017 and Forecast to 2023. Available from: https://www.reuters.com/brandfeatures/venture-capital/article?id=24054. Accessed on 30th August 2019 [Google Scholar]
[88]. Richards DJ, Tan Y, Jia J, Yao H, Mei Y, 3D printing for tissue engineering Israel Journal of Chemistry 2013 53(9-10):805-14.10.1002/ijch.201300086 [Google Scholar] [CrossRef]
[89]. Atala A, Forgacs G, Three-dimensional bioprinting in regenerative medicine: reality, hype, and future Stem cells translational medicine 2019 8(8):74410.1002/sctm.19-008931058436 [Google Scholar] [CrossRef] [PubMed]